In all sorts of animals, from fruit flies to mice to elephants, cells follow fairly similar sets of steps to grow from embryo to adult. But while these steps follow the same order and often involve the same kinds of genes and molecular signals, they proceed at different rates from species to species.
A mouse gestates in about 20 days; a human takes about nine months. An African elephant remains in the womb for nearly two years. And in step with their sizes — speaking broadly — goes the speed at which cells in their bodies develop.
So what are the timers that keep things trucking along at the right rate for any given organism, ensuring that it grows to the proper size and with all its parts in place? It’s a question that James Briscoe, a developmental biologist at the Francis Crick Institute in London, would love to answer.
Take one lone example among many: motor neurons, the nerves that make muscles contract. These develop from precursor cells over a few days in mice but a week or two in humans — and the same thing happens when the cells are grown in a dish. “We can look at this carefully and demonstrate it’s the same genetic process, the same gene activities, the same mechanisms involved, and it’s just running slower in humans than in mouse embryos,” Briscoe says. “We’re trying to tackle that problem.”
In a 2018 review published in the journal Development, Briscoe and developmental biologist Miki Ebisuya, now at EMBL Barcelona, explored where the field’s understanding of “developmental time” currently stands. It’s a two-part question, Briscoe explains.
First of all, for bodies to properly form, events must unfold in the right sequence: A before B, and B before C, and so on, at right times all over the developing body. Though there’s much still to know, scientists have amassed plenty of knowledge on this problem, especially (but not exclusively) in closely studied creatures like the roundworm Caenorhabditis elegans and the fruit fly Drosophila melanogaster.
For example, there’s a crisp order in how Drosophila neurons develop from stem cells in a part of the embryo called the ventral nerve cord. The stem cells produce neurons with different identities, one after the other; all of them develop, from the same pool of stem cells, just at different times, and once it’s time for the later types, there’s no going back to making earlier ones. They’re guided through this process by the sequential rise and fall of activity of a set of key genes.
The second aspect of timing is far more mysterious: the molecular processes setting the tempo such that clocks run faster or slower in different species.
What really counts?
Scientists already have identified types of clocks in sundry tissues. Speaking generally, such molecular timers either “count up by steadily increasing the levels of a critical regulator until it exceeds a threshold, or count down by gradually decreasing the levels of an inhibitor,” Ebisuya and Briscoe write.
Rats, for instance, use a “count up” timer to kick off brain-cell development in the embryo. As cells divide, chemicals that inhibit cell division gradually build up in the precursors to types of brain cells called oligodendrocytes. Once the inhibitors reach a key threshold level, the rat brain stops making new precursor cells and existing cells start to take on their mature forms.
In contrast, the African clawed frog Xenopus laevis employs a “count down” timer at a stage when that frog-to-be is a tiny ball of cells. Substances that spur cell division are diluted by speedy divisions until they’re so scarce that division slows and the next phase of embryo development kicks off.
Some clocks run through feedback loops, wherein proteins build up to a certain level and then act to shut down their production — creating cyclical oscillations that cells can harness to drive developmental steps. Vertebrates of all kinds depend on an oscillating clock of this type to create the right number of structures called somites, which later develop into the bones and muscles of the vertebral column. (For more on this segmentation clock, one of the best-studied examples of developmental timing, see this related article in our special report.)
And in other cases, cells seem to keep track of how many times they’ve divided, says developmental biologist Mubarak Hussain Syed of the University of New Mexico, who studies the timing of gene activity during Drosophila early brain development. “The cell might be counting, ‘OK, we have done 20 divisions, and now it’s time’ ” for the next step, he says.
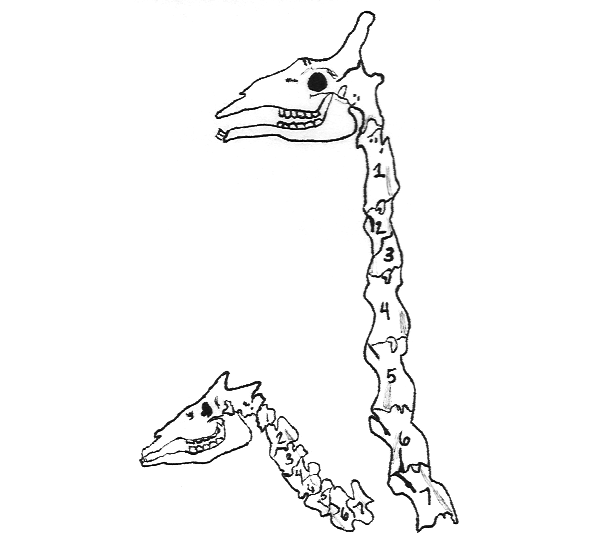
Despite their strikingly different neck lengths, the giraffe and its nearest relative, the okapi, both have the same number of neck bones, called cervical vertebrae. The vertebrae are merely longer in the giraffe. Scientists believe that changes in the timing of developmental events (slowing down growth of one part relative to another, for example) had a huge part to play in the evolution of species.
CREDIT: ASIANINVASION12 / WIKIMEDIA COMMONS
Whatever the mechanisms, external signals — not just clocks inside lone cells — are involved to keep everything in step. Drosophila larvae, for example, contain multiple clumps, or discs, of tissue that develop into structures of the adult fly: a pair of discs for the wings, another pair for the eyes, and so on. If one of the wing discs is damaged, scientists observe that development is delayed to give the disc time to repair.
Today’s modern technologies, including the ability to culture not just cells but mini-organs in dishes, should yield many clues. One might, for example, be able to add neuronal stem cells from one creature to a mini-brain of another and see if the foreign tissue develops at its own rate or changes its pace to match the cells that surround it, Syed says.
And the findings keep coming. Briscoe’s team recently posted unpublished results in the online archive BioRxiv suggesting that the difference in the pace of embryonic development of mice and humans may be caused by differences in protein stability between the two species. The differences appear to prolong the duration of the cell cycle in human cells.
Zooming in on details could yield a vast view. Scientists believe that changes in developmental timing — heterochronies, as they are called — had profound roles to play in the evolution of the diversity in body shapes and proportions we see in modern creatures. Snakes, for example, have many more vertebrae than do mice; they achieve this by running their segmentation clock at a faster clip relative to the development of other body parts. Giraffes come by their long necks another way: They have the same number of cervical vertebrae as their closest relatives, okapi, but those vertebrae are given more time to grow large.
By revealing the molecular clocks that time growth, biologists may start to understand the influences that gave the world mice, humans and elephants to begin with.