Since May 2015, in a portion of its WorldPort distribution center in Louisville, Kentucky, United Parcel Service has been operating a spare parts warehouse with no spare parts. Instead, the facility is stocked with ultrafast 3-D printers that can build up almost any plastic part that’s required, layer by layer by layer — and have it ready for UPS to deliver anywhere in the United States by morning.
“It was a no-brainer,” says Alan Amling, UPS’s vice president for corporate strategy. Storing spare parts for quick delivery was already a big moneymaker for the company, he says. UPS operates more than a thousand field stocking locations worldwide — all full of items that somebody might need someday, maybe. The industrial customers who pay for that service have to keep the parts available because of warranty contracts, says Amling. But they hate it. “Inventory storage costs are massive,” he says. “So we started to see 3-D printing as a solution.”
Although 3-D printing isn’t cost-effective for every part, says Amling, the Louisville microfactory has already been successful enough for UPS to replicate it at several sites around the United States, with plans to eventually take it worldwide. And that’s before the centers add the capacity to print metal parts later in 2018. But what Amling particularly savors is the way 3-D printing has blurred the boundaries. When a delivery firm suddenly starts doubling as a manufacturer, he says, you’ve arrived at someplace new — “a white space.”
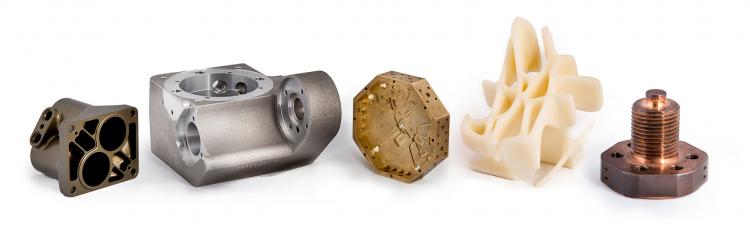
As part of an effort to supply parts "on-demand," United Parcel Service has partnered with Fast Radius, which can tap an array of additive and traditional methods to custom build metal and plastic parts (shown).
CREDIT: UPS
It’s the kind of genre-scrambling that 3-D printing is starting to get very good at. The various technologies that fall under that heading have always encompassed much more than the low-cost hobbyist machines most of us know. But in just the past few years, 3-D printers have begun to emerge as a serious alternative to standard fabrication techniques like casting, milling and injection molding. Often called “additive manufacturing” for the way they add material to make a part rather than grinding it away, this suite of 3-D technologies is increasingly being seen as a force that could transform the very idea of a factory, in much the same way that personal computers have transformed the rest of the world.
“Additive has lots of hype. But take 90 percent of that hype and throw it away, and the rest is still revolutionary.”
Mike Molnar“I think of additive as the democratization of manufacturing,” says Mike Molnar, a manufacturing engineer turned policy director at the National Institute of Standards and Technology in Gaithersburg, Maryland. Molnar directs Manufacturing USA (initially called the National Network for Manufacturing Innovation), a series of federally funded laboratories that do research on additive technologies, artificial intelligence, robotics and other advanced industrial techniques.
As he explains it, 3-D printing promises a new era of on-demand, distributed manufacturing that’s as close as possible to the consumer — when it’s not literally in the hands of the consumer. UPS’s Louisville microfactory is an example. So is the 3-D printer that NASA installed on the International Space Station in March 2016, so that astronauts can print hard-plastic ratchet wrenches and other parts without having to wait months for the next resupply launch. In neither case does anyone need elaborate assembly lines and machine tools, just digital files.
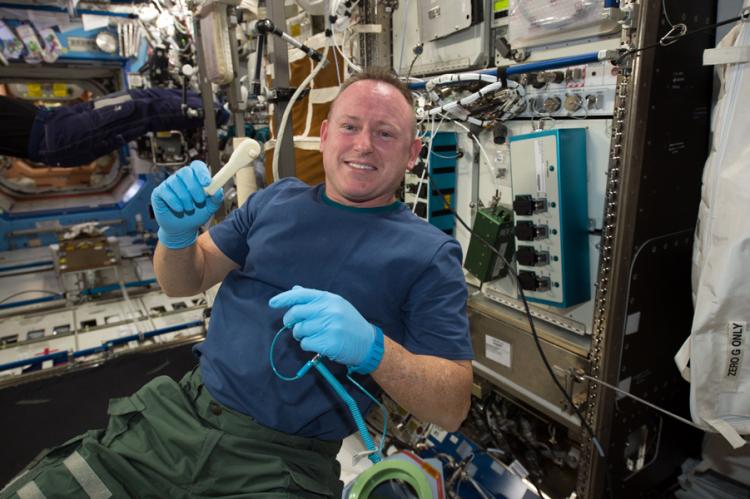
A part of 3-D printing's appeal is its ability to create tools and parts as needed, in remote locations. Aboard the International Space Station in 2014, NASA astronaut Barry Wilmore displays a ratchet wrench created from a 3-D printer a long way from Earth.
CREDIT: NASA
But just as important, says Molnar, 3-D printing unleashes the creativity of inventors and designers. They can optimize their parts for shape, strength, weight or whatever else they can imagine, without ever having to worry about whether it’s physically possible to machine, cast or assemble the things. One example is the SuperDraco thruster developed by the private rocket company SpaceX. The intricately shaped nickel-alloy device isn’t put together in the usual way from dozens of smaller parts. It is 3-D-printed as a whole from metal powder fused by a laser. Another example is Adidas’s Futurecraft 4D running shoe line, which features midsoles with a 3-D-printed foam structure that is designed for ideal support and minimal weight — and that can be tailored to each customer’s foot and running style.
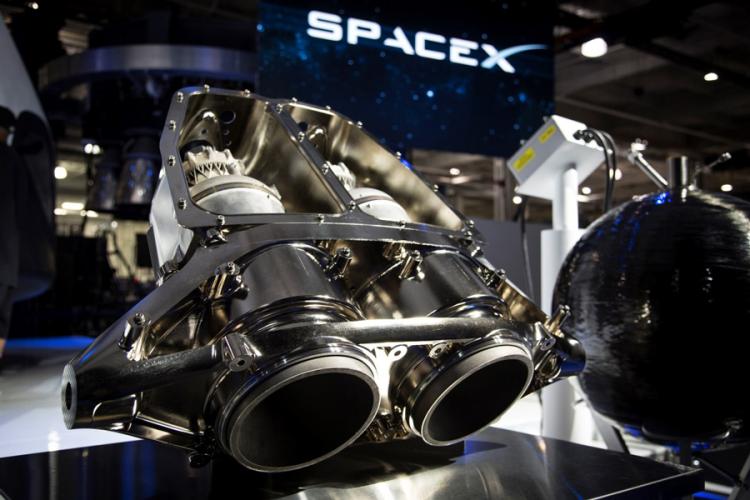
SpaceX launched its first metal rocket hardware created by 3-D printing in 2014. The SuperDraco Thruster, shown here, is made of a high-performance metal alloy and was created using additive manufacturing techniques in less time than traditional machining. The thruster was designed to fire as part of the launch escape system for SpaceX's piloted Dragon spacecraft.
CREDIT: SpaceX
Granted, says Molnar, “additive has lots of hype.” In the real world, for example, most additive technologies are much slower than standard fabrication methods like casting or machining, and can work with only a comparative handful of polymers, ceramics and metal alloys. “But take 90 percent of that hype and throw it away,” says Molnar, “and the rest is still revolutionary.”
That prospect is why the Obama Administration made additive technology a top priority when it launched Molnar’s manufacturing research program in 2011. It’s why established companies and start-ups alike have entered the game, determined to achieve dramatic increases in printing speed. In 2016, for example, HP Inc. (formerly known as Hewlett Packard) introduced a system that can churn out plastic parts ten times faster than previous 3-D printers at half the cost. The machines can even help “print the printer” by making some of the parts required to clone themselves.
And it’s why companies like the German firm EOS, which has been making 3-D printers for nearly three decades (including the ones used by SpaceX), is experiencing a boom in demand. “It took us twenty years to sell our first 1,000 printers,” says Glynn Fletcher, president of EOS’s North American division. “Today we’re gearing up for 1,000 per year.”
“Additive manufacturing today is a $5- to $6-billion industry,” continues Fletcher. And while that’s a lot, he says, “it’s only a tiny fraction of 1 percent of the total manufacturing industry.” So with even a little bit of additional market penetration, “additive has the potential to go from $5 billion to $50 billion in the not too distant future.”
“We have the potential to be very disruptive,” says Fletcher. “We just have to figure out how.”
Homebrews
The idea of 3-D printing was definitely in the air by the early 1980s, says Timothy Gornet, who manages the Rapid Prototyping Center at the University of Louisville in Kentucky. “Laser and inkjet 2-D printing was advancing rapidly,” he says, “so I believe for engineers it was natural to think, ‘If I can drive a 2-D printer from a digital file, why not do that to create a 3-D part?’”
In fact, he says, that idea was so compelling that inventors independently found more than half a dozen ways to implement it.
One evening in March 1983, for example, engineer Charles Hull phoned home from the little lab his company let him use nights and weekends. Come on down here, he told his wife — you’ve got to see this!
“It had better be good,” replied his wife, who was already in her pajamas.
It was. Hull’s day job was to make plastic coatings for tabletops: Just brush on a liquid acrylic resin, hit it with ultraviolet light, and the resin would instantly harden into a protective surface. But on his own time, Hull had been trying to add a dimension. His idea was to take a pot of that same resin, illuminate its surface with ultraviolet light in a pattern that would make a portion of the liquid harden into the cross-section of some part, then raise the level of the liquid a tiny bit to form a new layer and repeat. Eventually, he would be able to drain the unhardened liquid to reveal the finished item.
Hull called the process “stereolithography” — a mashup of Greek terms that roughly translates as “3-D writing in stone.” What he proudly showed his wife on that March evening was his first successful print job: a small plastic eye cup that he still has. The company Hull cofounded in 1986 to commercialize the technology, 3D Systems of Rock Hill, South Carolina (originally based in Valencia, California), continues to be a major player in the field.
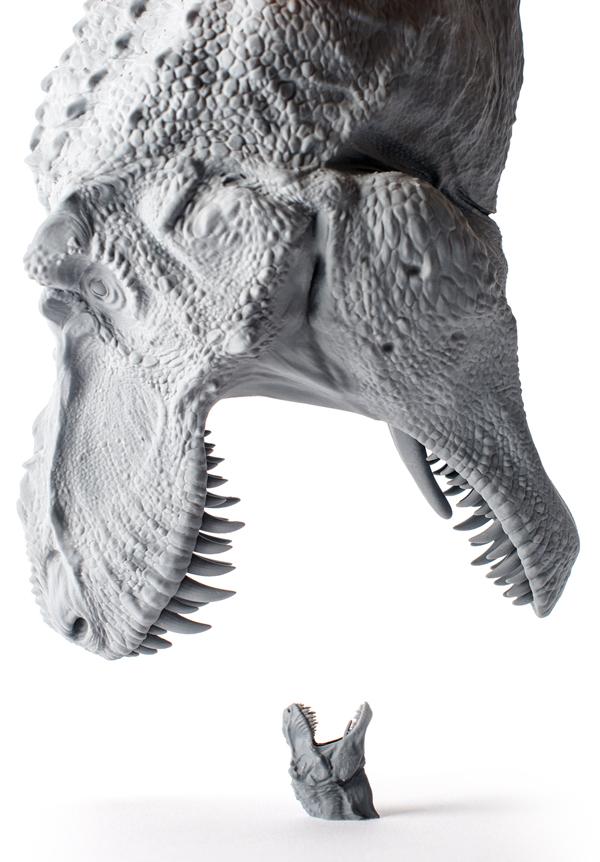
Plastic T. rex toys printed by Carbon using a variant of stereolithography.
CREDIT: Courtesy of Carbon
Three years later, in Eden Prairie, Minnesota, mechanical engineer Scott Crump carried out an experiment in the family kitchen. He’d been inspired by the way standard plotters move pens around on 2-D sheets of paper to make blueprints and architectural drawings. He wanted to do something similar in 3-D, using “pens” that built up solid objects by extruding material one layer at a time. And he’d finally figured out a way to demonstrate the idea. Using a handheld hot-glue gun that he’d filled with a mix of polyethylene and candle wax, he systematically built up a toy plastic frog for his 2-year-old daughter.
She loved it. And that positive customer response encouraged Crump to keep experimenting with the technique, which is now called fused deposition modeling (FDM). His wife, Lisa, finally exiled him from the kitchen to the garage after everything the family ate started tasting like plastic. But Stratasys, the company that Scott and Lisa Crump founded later in 1989, has gone on to become another major maker of 3-D printers.
At the University of Texas in Austin, meanwhile, a mechanical engineering graduate student named Carl Deckard had spent the mid-1980s working out the kinks in a scheme that had obsessed him since his senior year in college. The idea was to lay down a thin layer of powdered plastic — Deckard initially used a large salt shaker — then trace the cross-section of a part with a laser guided by a computer (a heavily modified Commodore 64.) The plastic would melt wherever the laser touched it, fusing with the cross-section below. Then the process would repeat after he added a new layer of powder. At the end, the leftover powder could be vacuumed up for reuse, leaving just the finished part.
Deckard eventually got this laser sintering technique to work well enough to earn his master’s degree in 1986 and his PhD in 1988. The company he cofounded to commercialize it, DTM, was later purchased by 3D Systems — and laser sintering is now one of the go-to techniques for industrial-strength additive manufacturing.
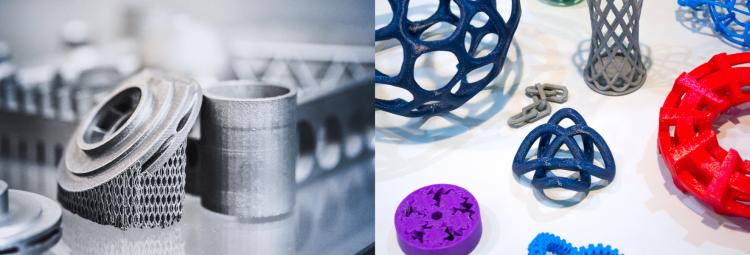
Additive manufacturing allows the creation of intricate designs in a variety of shapes and sizes. Although plastics, right, have been the most well-known products of 3-D printers, the last decade has brought progress in making metal parts, left, as a laser sintering process and other methods opened up new vistas for the technology. Challenges remain in speed, materials and infrastructure.
CREDIT: MARINA GRIGORIVNA / SHUTTERSTOCK
In the early years, these 3-D printing techniques and the others that soon followed were collectively known as “rapid prototyping” — largely because that was almost the only profitable application. 3-D printing turned out to be a very good way to make new device prototypes, which engineers still liked to use for checking fit and function, or for holding in their hands as a communication tool. (“If a picture is worth a thousand words,” says Gornet, “a part is worth a thousand pictures.”)
The pioneers in the field had plenty of other applications in mind, adds Gornet. “As soon as engineers were able to get prototypes quickly,” he says, “they immediately started to think how 3-D printing could be used” to advance manufacturing customization, on-demand spare parts and all the rest. But reality too often intervened. Early 3-D polymer parts were often quite brittle, for example. They also tended to be expensive, mainly because the printing was so painfully slow that it took days to make even simple parts. And the range of available materials was quite limited, with metal and ceramic parts not yet out of the laboratory, and polymer parts pretty much restricted to nylon.
Still, by the end of the 1990s researchers had made steady progress on these problems, and 3-D printing was beginning to find other profitable niches in applications where the advantages outweighed the time and cost penalties. Orthodonture was an early example: In 1999, the Netherlands-based firm Align Technology introduced Invisalign, first of the removable, clear-plastic inserts that serve as an alternative to conventional wire braces. Because each of the devices had to be custom-fitted to an individual patient’s teeth, Align started 3-D-printing them with stereolithography, as did the competitors that followed. This meant that the inserts were roughly twice as expensive as wire braces — the cost of a full set today averages $5,000 — but the convenience factor made them a hit anyway. They require fewer orthodontist visits, and can easily be removed for eating and tooth-brushing. The industry is currently printing millions of the inserts per year, each of them customized by a simple change in the printer’s programming.
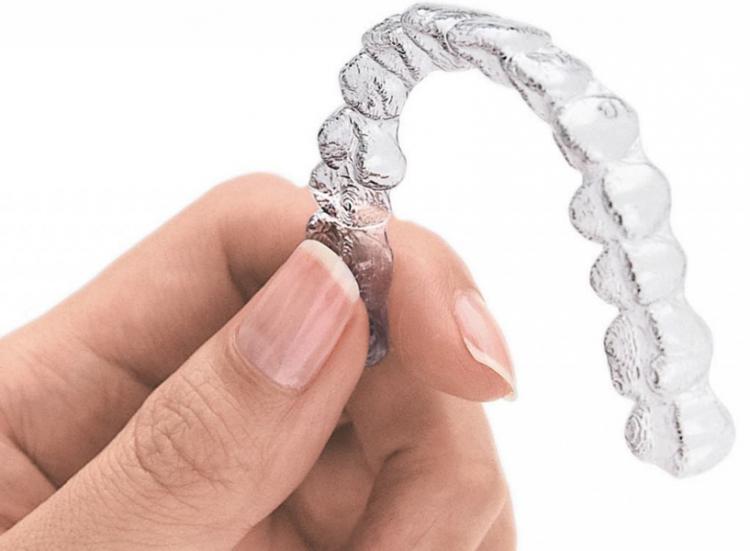
One of the most commercially successful applications of 3-D printing so far has been customized, see-through braces (sold as Invisalign and under other names) made by stereolithography.
CREDIT: DAVIS DENTAL / WIKIMEDIA COMMONS
Hearing aids followed a similar path, starting in 2000 when the Swiss company Phonak began using stereolithography to make hearing aids that were customized to fit each patient’s ear canals. Today, the vast majority of in-ear hearing aids are made this way.
Tipping point
A few years into this century, the industry reached a tipping point, as sales of all kinds of 3-D fabricators and raw materials began to accelerate substantially. One impetus was simply the passage of time: Because US patents generally last for only 20 years, the ones granted during that first flurry of 3-D printing innovation in the 1980s were beginning to expire. This had a particularly strong impact on the low-end hobbyist market, which was (and is) almost entirely based on fused deposition modeling — Crump’s glue-gun extrusion technique. The raw materials were inexpensive and nontoxic, and the fabricators were safe to use in the home or office. So after Crump’s basic patent expired in 2009, copycats were quick to jump in, the price of the machines fell from more than $10,000 to under $1,000, and they became common sights in “makerspaces” and even schools. “I once counted about 50 companies marketing low-end 3-D printers,” says University of Texas materials engineer David Bourell.
Another key factor was that laser sintering of metal parts had finally emerged from the laboratory, after years of efforts by Bourell and others to eliminate voids and weaknesses in the fused powder grains. EOS announced the first commercial metal fabricator in 2004, and continues to dominate this sector. “The metals machines offered a way to manufacture complex parts and achieve mechanical properties very similar to those from traditional methods,” says Gornet. And this, he says, “really triggered the thought that additive manufacturing may be able to grow dramatically” — that 3-D printing could move out of its niche markets and begin to challenge traditional technologies on their own turf.
For that to happen, however, researchers would have to overcome 3-D printing’s biggest shortcomings, starting with its painful slowness. Using standard laser sintering, says Amling, “it would take two days to make a coffee cup.”
That slowness automatically puts additive manufacturing at a severe economic disadvantage, says John Dulchinos, vice president for global automation at Jabil, an international “contract manufacturing” company that does much of the actual production for brand-name firms such as HP. For most products, says Dulchinos, manufacturers typically do production runs numbering in the hundreds of thousands. But today, he says, “3-D is competitive only at tens of thousands.” And even that is only when the parts are intricately shaped and small. When you’re building things up point by point, notes Bourell, “if a part is twice as big, it takes eight times longer.” All of which is why researchers in industry and academia alike have been making speed a top priority.
One notable effort is HP’s first foray into 3-D printing, which has drawn a lot of attention because the company is already a major player, not just another start-up. The company’s “Multi Jet Fusion” fabricator first lays down a thin layer of powdered polymer in much the same way as a laser sintering system. But instead of tracing the cross-section of the part with a laser, it prints the cross-section with microscopic drops of infrared-sensitive ink. (“The print heads are the same as those we use in our industrial inkjet printers,” says Paul Benning, HP’s chief technologist for 3-D printing.) Then it follows up with a flash of heat that is absorbed by the ink, which fuses the powder underneath. Finally, the cycle repeats with a new layer.
This all happens very quickly, making HP’s machine about 10 times faster and substantially cheaper than an equivalent laser sintering system. “HP is pretty good at inkjets,” notes Bourell. That said, the first model is limited to plastic parts; the company has announced that metal fabricators are coming, but not until later in 2018.
And by that point, HP may well have company: Desktop Metal, a startup based in Burlington, Massachusetts, has announced that it will soon be coming out with a high-speed metal fabricator that will work in somewhat the same way as HP’s. (Instead of ink, it sprays each layer with a binder that holds the powder together until the whole part can be fused in a separate heater.) “Our study concluded that you can do well over 500 parts in a day, while with lasers you could do 12 in a day,” says Desktop Metal’s chief technology officer, Jonah Meyerberg. The company’s machines can also use a wider (and cheaper) range of metal powders, he says, since the material doesn’t have to be optimized for laser melting.
In Redwood City, California, meanwhile, Carbon, Inc. has achieved dramatic speed-ups in stereolithography. Carbon founder and president Joseph DeSimone credits several key innovations. The first, developed in his lab at the University of North Carolina, Chapel Hill, is a technique that exposes the working liquid to ultraviolet light and oxygen simultaneously. The resulting chemical interplay, dubbed Continuous Liquid Interface Production (CLIP), “allows us to go really fast” in printing an object, says DeSimone — up to 100 times faster than conventional stereolithography. That’s one reason Adidas is using the company’s fabricators to make its Futurecraft 4D midsoles.
The second innovation is that the working liquid isn’t just a single photosensitive resin, but a mix of several compounds. “Only about 10 to 15 percent of the volume is light sensitive,” says DeSimone, and is there simply to set the shape of the part. The other components are chemicals that will react to form a strong polymer once the fully shaped part is sent to a baking oven. “It’s like epoxy glue, where you get the bond when you react two liquids,” he says. This mixing approach also allows the company to produce a much wider range of materials than was possible with earlier stereolithography systems.
Expanding the range of available materials is, in fact, another key challenge for everyone in the field. EOS, for example, is taking a comparatively incremental approach to increased speed — mainly by improving the fabricators’ powder-handling, and having two, four or even eight lasers working in parallel. But the company has a whole subsidiary, Advanced Laser Materials (ALM), working to improve the stuff that goes under the laser.
This is especially difficult when it comes to polymers, says ALM President Donnie Vanelli. Within the laser sintering sector, he says, “80 to 90 percent of what’s moved to date has been built on nylon.” That’s because nylon is one of the few polymers that can not only be ground into a powder that rolls out nicely in the machine, but also melts well under the laser. ALM has been able to make nylon harder, stiffer, lighter and stronger with fillers such as carbon fiber, tiny glass spheres and even aluminum granules. “But nylon is not what people typically build parts out of,” says Vanelli. “So, if we really want to expand the future in additive, we have to be able to tell engineers, ‘We can do the materials you’re familiar with.’” There’s been some progress. In November 2017, for example, EOS and sportswear maker Under Armour announced a partnership to develop running shoes with soles made by laser-sintering a flexible polyurethane that’s very common in footwear.
The fundamental challenge
For everyone in additive manufacturing, however, easily the biggest challenge is what EOS’s Fletcher calls “the habits of the present.”
His office is in Michigan, explains Fletcher, surrounded by factories supplying parts to the automotive industry. “They’re making things in the traditional way,” he says. “They are comfortable with that. And they are not going to overnight throw all that equipment in the dumpster and go to additive manufacturing.”
On the other hand, he says, when you talk to engineers about the advantages of additive — the nonexistent retooling costs, say, or the freedom to design parts for function instead of for manufacturability — “everybody gets that.” So he thinks it’s a matter of when people make the jump, not if.
Certainly it’s the case that EOS, like HP and most other players in the additive manufacturing field, finds itself hosting a growing stream of potential customers who are intrigued by the possibilities — and daunted by the learning curve. “This is a technology that’s very disruptive to process engineering and design,” says EOS marketing director Patrick Boyd. “Potential customers come to us and ask, ‘How do we even start?’” By 2016, he says, EOS was hearing that question so frequently that it launched Additive Minds: a dedicated training division with 100 instructors in five centers worldwide. “It’s Additive Minds’ job to tell people about how to select applications, who needs to be trained, where are the early adopters succeeding,” says Boyd.
It seems to be working. In one quarter of 2017, he says, the company sold more than it did in all of 2016. Indeed, the potential looks strong for additive manufacturing across the board.
“We’re at the middle of the beginning,” says Boyd, “and it’s going to ramp up very quickly.”
Editor's note: This article was updated on May 15 to reflect the current name of HP Inc., which the article originally referred to as Hewlett Packard.