Spots, stripes and more: Working out the logic of animal patterns
More than 70 years ago, mathematician Alan Turing proposed a mechanism that explained how patterns could emerge from bland uniformity. Scientists are still using his model — and adding new twists — to gain a deeper understanding of animal markings.
Support sound science and smart stories
Help us make scientific knowledge accessible to all
Donate today
There’s a reason fashion designers look to animal prints for inspiration. Creatures have evolved a dizzying array of patterns: stripes, spots, diamonds, chevrons, hexagons and even mazelike designs. Some, like peacocks, want to be seen, to attract a mate or scare off a rival or predator. Others, like tigers or female ducks, need to blend in, either to sneak up on prey or to avoid becoming lunch themselves.
Some patterns arise simply or randomly, but others develop via complex, precise interactions of pattern-generating systems. Their beauty aside, the intricacies of these systems are inspiring the scientists who aim to elucidate how the tiger got its stripes, the cheetah its spots and more besides.
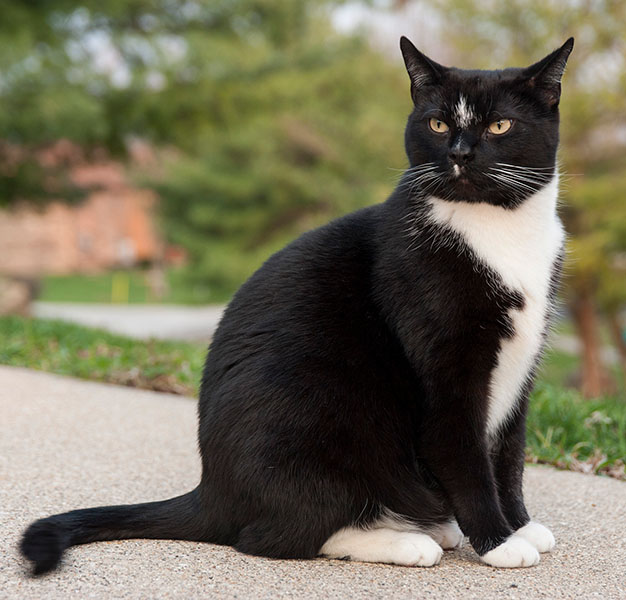
Tuxedo cat with black fur and white belly.
CREDIT: ADKISAAC / WIKIMEDIA COMMONS
Mammals like cats and dogs can have white tummies. They get them in a straightforward way: As the embryo develops, pigment-making cells originate along the site of the future spine and migrate down and around toward the belly. But sometimes they don’t make it all the way. Where the pigment cells run out of steam, the white begins.
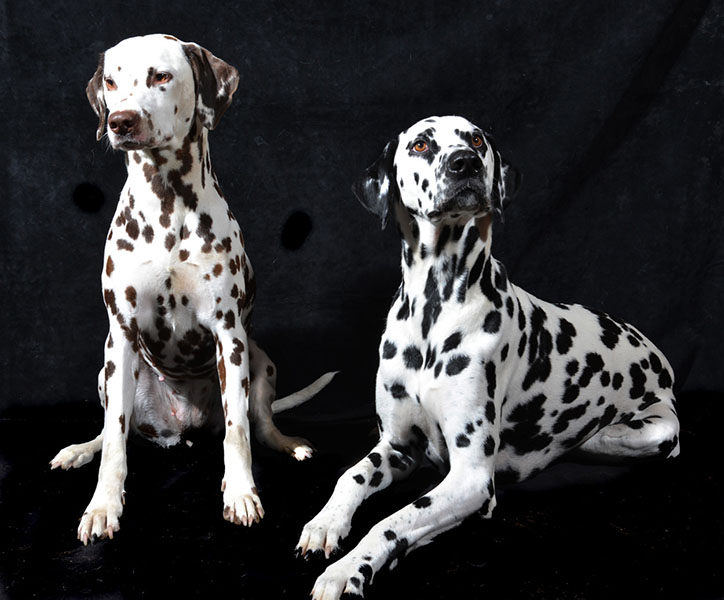
Two handsome, very spotty dalmatians.
CREDIT: PHILIP WATTS / FLICKR
The black dots on Dalmatians are generated randomly. So are the black-and-orange splotches on calico cats.
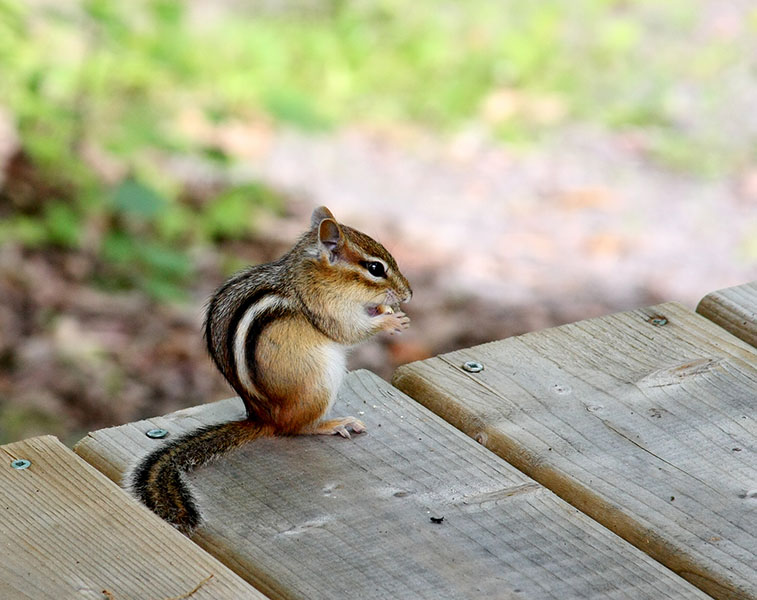
An eastern chipmunk snacking.
CREDIT: PAUL HARRISON / WIKIMEDIA COMMONS
But the stripes of chipmunks and tigers, the speckles on fishes and chickens, and many other glorious animal features are laid down with exquisite precision. In a remarkable feat of self-organization, a uniform surface becomes patterned.
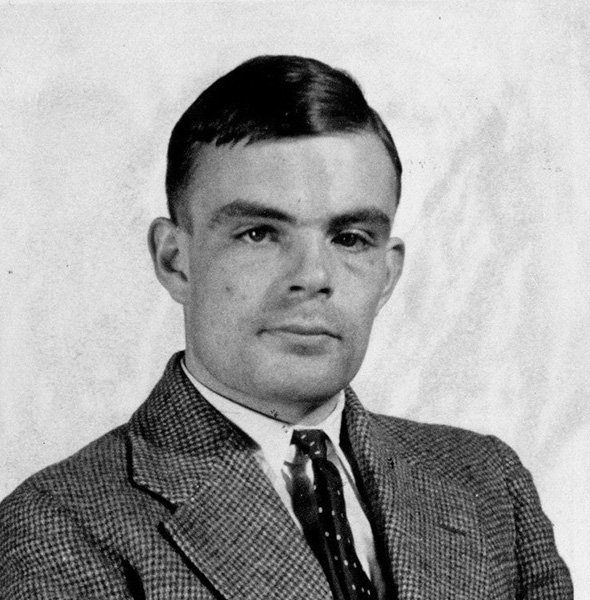
Alan Turing (1912-1954).
CREDIT: WIKIMEDIA COMMONS
The person who figured out how this happens was Alan Turing. You may know him as the 20th century mathematician who broke Nazi codes during World War II and developed early concepts in artificial intelligence. Turing also turned his math skills to understanding how regular features could emerge on the developing embryo. Scientists since then have applied his equations to the development of such patterns as fingerprint ridges, the places where hairs will sprout, and color patterns like stripes and spots. And it turns out he was really onto something: Today, scientists studying animal patterns still find Turing’s ideas to be remarkably effective — especially when combined with other factors that elaborate the patterns further.
Here’s a colorful tour of what scientists are learning today, beginning with Turing’s classic theory.
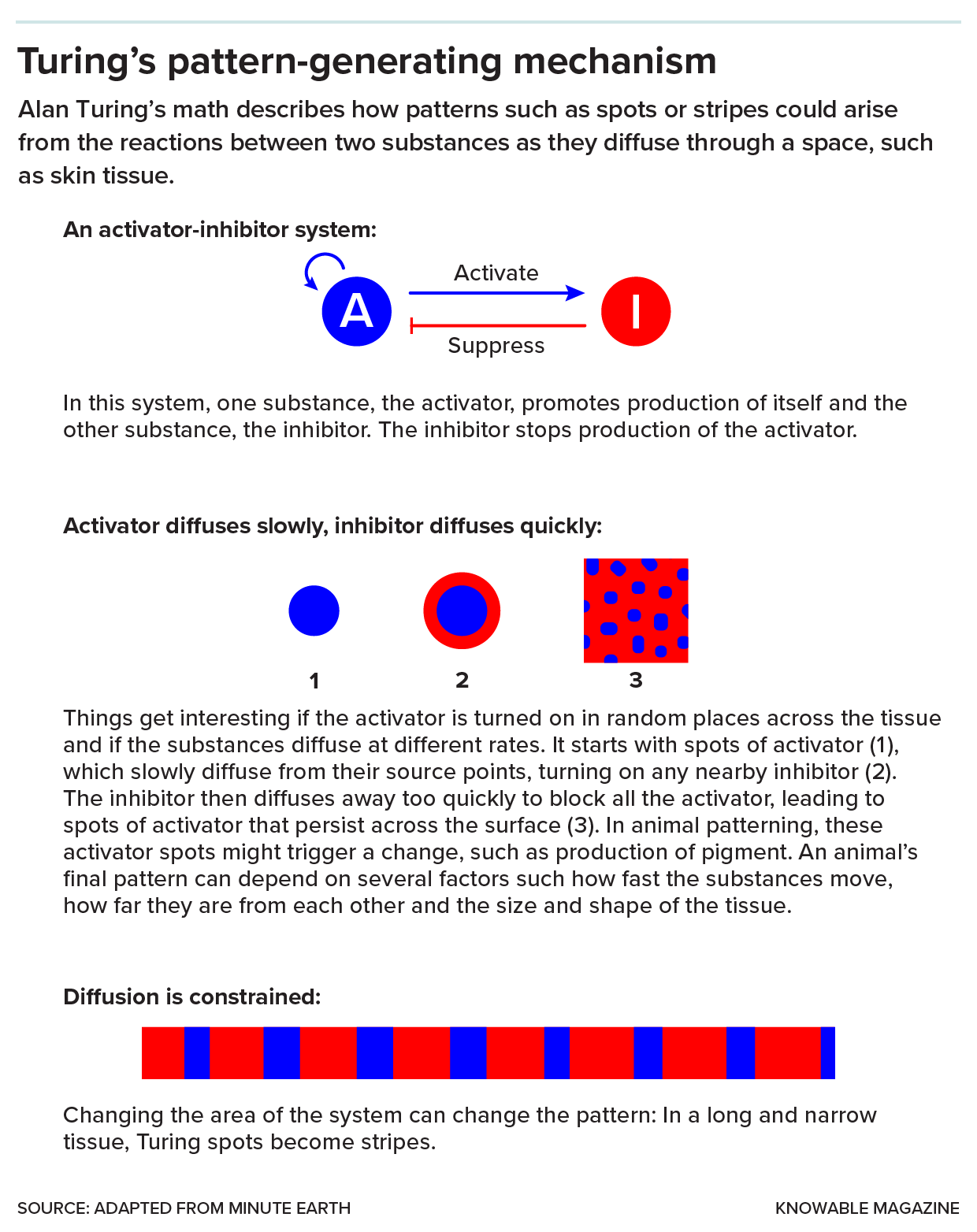
Turing’s ideas explain how some animal patterns arise.
A spot-maker is the most basic version of Turing patterning. It involves two key substances, or morphogens, as Turing called them, that can move through the developing skin. One substance, the activator, turns itself on and also turns on the other substance, the inhibitor. The inhibitor blocks the activator.
By itself, that system doesn’t do much. But if the substances diffuse through tissues at different speeds and some random fluctuations are introduced, it can yield a pattern of stable spots on fur, feather or scale. Say the activator is randomly turned on in various places — it diffuses from its source, turning on more of itself and the inhibitor as it moves. If the inhibitor diffuses faster than the activator, there won’t be enough locally to block all the activator activity. This can result in stable, evenly spaced activator spots surrounded by zones of inhibitor.
Changing the parameters of the system, such as how quickly the morphogens are generated or travel, or the size and shape of the space in which they move, can alter the final pattern. For example, a cheetah’s tail is long and skinny; in that narrow space, the spots coalesce into stripes. “A simple mechanism can create an amazing, diverse and rich variety of patterns,” says Seita Miyazawa, an evolutionary biologist at Osaka University in Japan.
Way to go, Alan.
Watch this video for a more in-depth explanation of Turing patterning.
CREDIT: MINUTE EARTH
But sometimes Turing’s ideas alone aren’t sufficient to explain nature’s magnificent patterns. Scientists must invoke additional players. Rather than simple diffusing chemicals, cells themselves may get in on the act. Or animals might need additional tricks to transport the morphogens across tissues, or to make patterns sharp and crisp. Scientists also see dizzyingly complex instances where Turing patterns are overlapped with additional patterning mechanisms or have more than just two interacting morphogens.
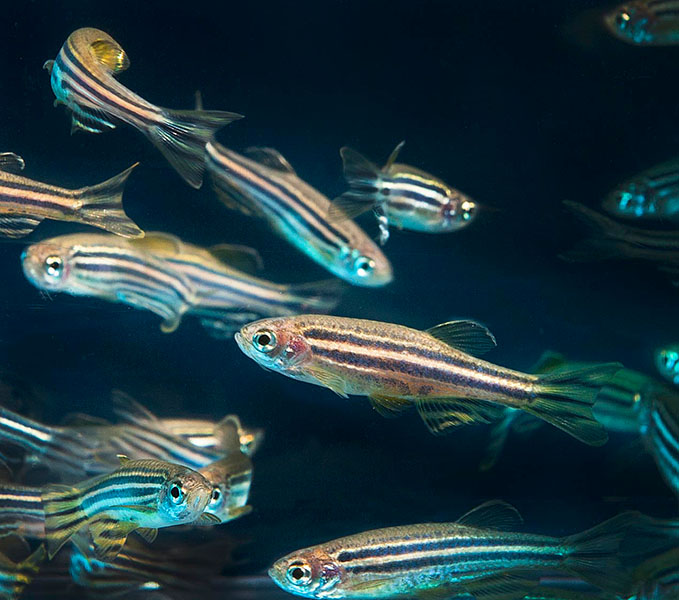
Developmental biologists have worked out some, but not all, of the details behind zebrafish striping.
CREDIT: OREGON STATE UNIVERSITY / WIKIMEDIA COMMONS
The zebrafish, one of developmental biologists’ favorite workhorses, sports clean, black-and-yellow stripes from head to tailfin. In this case, it’s not so much diffusing substances that create this pattern, but more complex interactions between the pigment cells themselves. The cells come in two key types: black melanophores and yellow xanthophores. At short range, they kill or repel each other, a rivalry that separates them into distinct stripes along the fish body. But at the same time, the black cells will die if they don’t receive some substance, as yet unidentified, from the yellow cells. Thus, they linger at a safe distance.
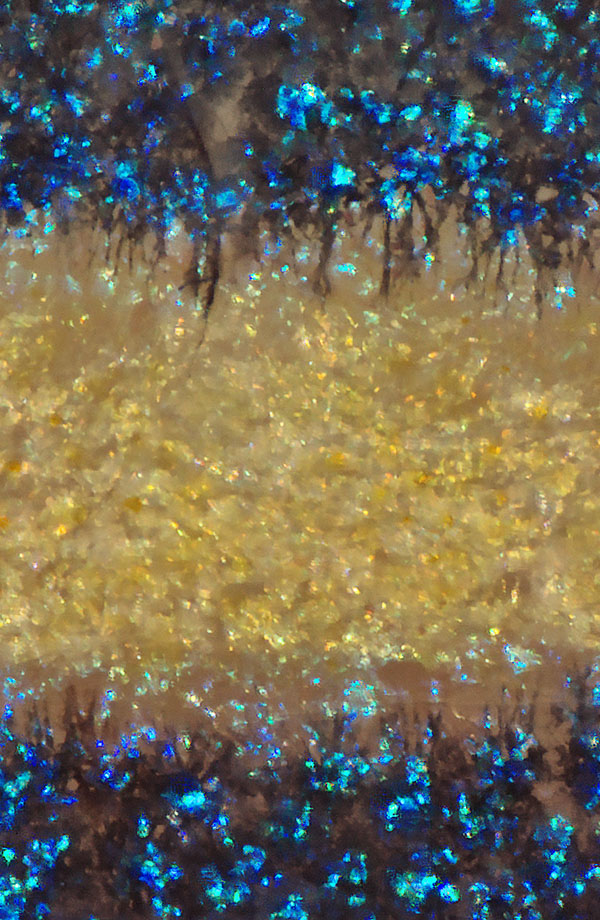
The black cells of a zebrafish extend projections to obtain some necessary substance from its yellow cells.
CREDIT: L.B. PATTERSON & D.M. PARICHY / AR GENETICS 2019
By Turing’s theory, the substance the black cells need from the yellow cells would arrive via diffusion, but there’s a problem, says Cheng-Ming Chuong, a biologist at the University of Southern California. The yellow-cell stuff would have to cross molecularly vast distances — more than 200 micrometers, or the length of about 20 cells — to reach the black cells. That’s just too far for diffusion to be efficient. Scientists found the zebrafish’s solution in long, skinny appendages that the black cells extend into the yellow areas, like arms reaching for that necessary substance. And it turns out that when the stripes are first forming, the developing yellow cells also make projections toward black cells, delivering another mysterious factor that pushes the black cells together into stripes.
That’s all cool, but it only explains how the cells minimize the distances those special substances have to travel, not how the goods get from one cell to the other.
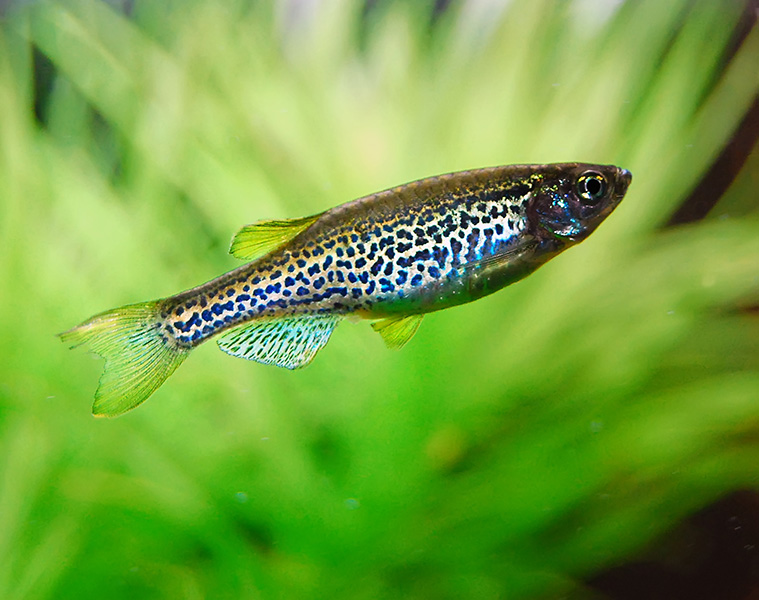
Mutant zebrafish can have spots instead of stripes.
CREDIT: CAROLINECCB / FLICKR
This oddball zebrafish suggested an answer. It’s a mutant version of the fish, called “leopard” because it has spots instead of stripes. The gene that’s broken in the mutant is involved in making little channels, called gap junctions, between cells. So it may be that the fish needs not just long cellular limbs but also gap junctions to move the substances that create the stripes.
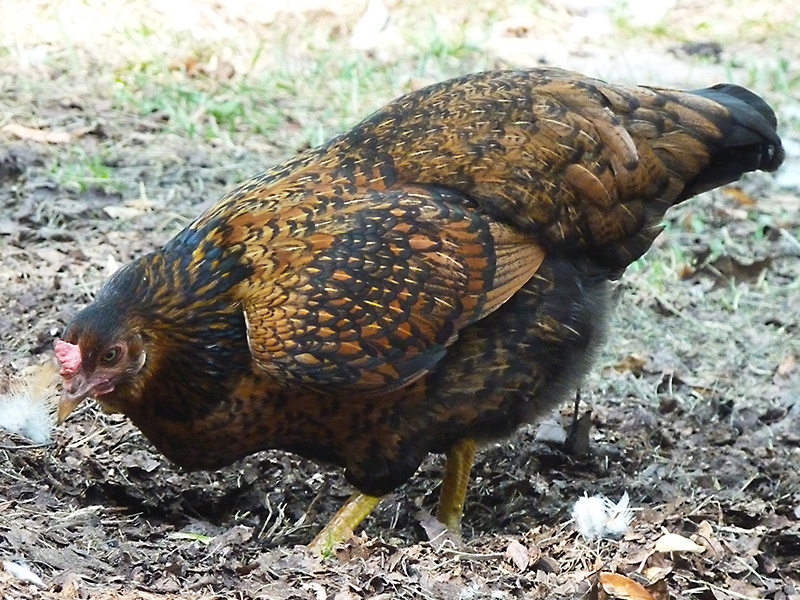
A Dark Cornish mix chicken scratches away.
CREDIT: NORMANACK / FLICKR
Some birds also seem to use skinny cellular projections and gap junctions in their patterning. Chuong and colleagues have found that both features are involved in the head-to-tail stripes in Japanese quail. When the researchers grew quail skin in a dish, visible yellow and black stripes formed, but the yellow stripes got very skinny when the gap junctions were shut down with a chemical inhibitor. Gap junctions also contribute to the complex feather striping mutation known as Melanotic in chickens. Leif Andersson, a geneticist at Uppsala University in Sweden and coauthor on the chicken study, thinks there may be some unknown morphogen that travels — or fails to travel — through the gap junctions to create the feather patterns.
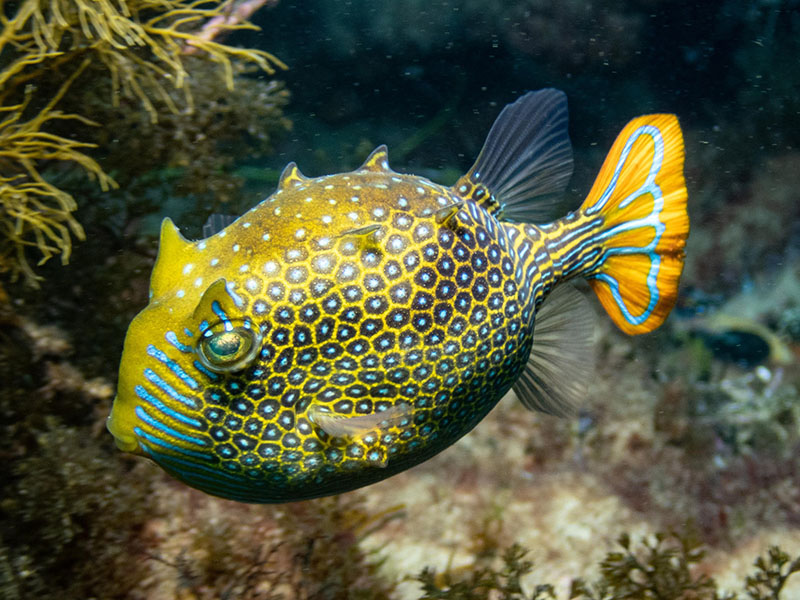
An ornate boxfish has remarkably crisp definition of its patterning.
CREDIT: imogenisunderwater / iNATURALIST
The ornate boxfish with its crisp hexagons seems to have its own solution to the diffusion problem. Presumably, if the morphogens that control its pattern had to diffuse across tissues, they couldn’t create such neat, angular lines. Think of a dye spreading in a thick liquid: Droplets of different colors would eventually become fuzzy blobs.
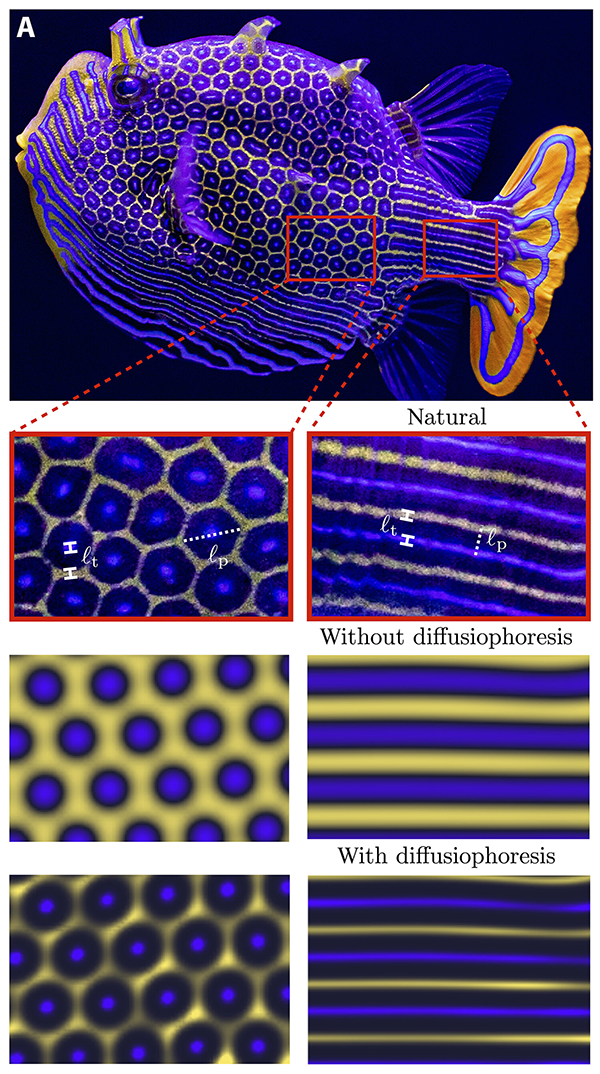
A boxfish (top row) exhibits crisp patterning (second row). Computer models to create the same pattern come out blurry (third row), but including diffusiophoresis (bottom row) improves the resolution.
CREDIT: B.M. ALESSIO & A. GUPTA / SCIENCE ADVANCES 2023
Fuzzy patterns were just what resulted when chemical and biological engineer Ankur Gupta at the University of Colorado, Boulder, and his then research assistant Ben Alessio tried to simulate hexagonal patterns in a computer Turing model. They were nothing like what nature produces. But the scientists found a solution in a concept called diffusiophoresis, in which small molecules push or pull bigger ones; it’s how small soap molecules yank big bits of dirt from your clothes in the washing machine. When the researchers added diffusiophoresis to their models, the patterns looked a lot more like the boxfish’s scales, though they were still far from perfect.
The researchers suspect that some small Turing morphogen is dragging the fish’s pigment cells into place, and that the fish uses other patterning methods, too. “Absolutely, there are other factors that might be at play,” says Gupta.
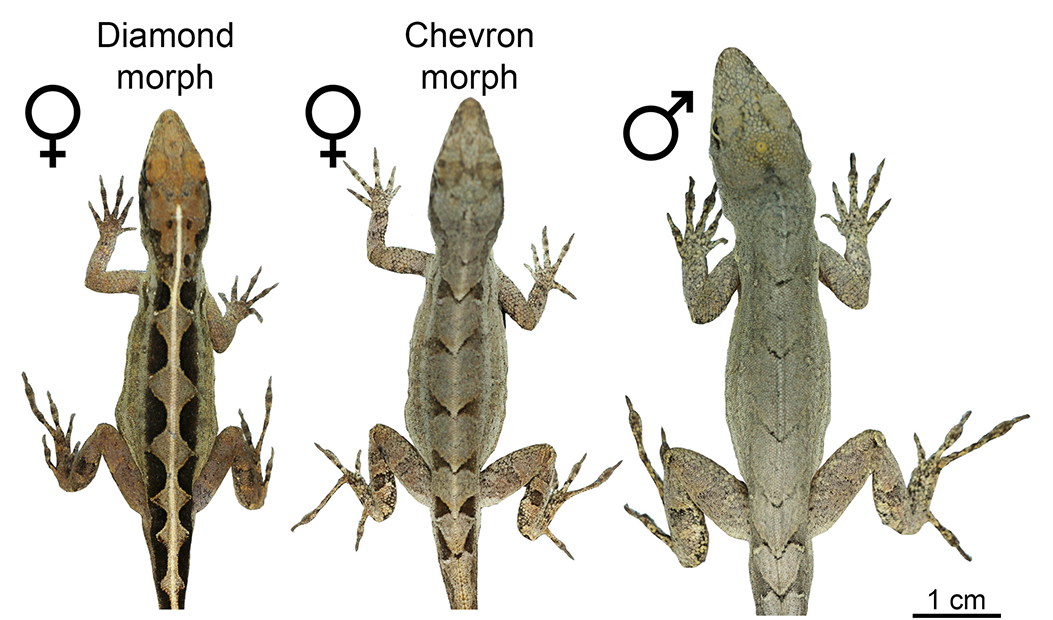
Female brown anoles come in diamond or chevron versions, but males always sport chevrons.
CREDIT: N. FEINER ET AL / SCIENCE ADVANCES 2022
The brown anoles found throughout the Caribbean created a different sort of Turing puzzle for Nathalie Feiner, an evolutionary biologist at Lund University in Sweden. All the males appear to use the same tailor: They have dark chevrons all down their backs. But females come in two fashions: chevrons like the boys, or a pattern of light diamonds flanked by darker triangles. Feiner thinks that a Turing-type pattern could easily explain the diamonds, with cells of different colors migrating outward from the site where the spine will eventually form. But with the chevrons, it looks like those diamonds are smearing toward the tail. Why so?
Genetics often provides clues to patterning mechanisms, and Feiner discovered the roots of anole fashion in a gene called CCDC170. One version of the CCDC170 gene generates diamonds, and another chevrons. The diamonds dominate, so any female with at least one diamond version will be a diamondback. But it happens that females make more CCDC170 protein overall than males. Thus, even if males have the diamond version of the gene, they don’t seem to be able to muster up a diamond pattern.
The function of the CCDC170 protein also provided a clue: It affects how cells move around. The scientists don’t understand exactly how different versions of the gene change patterning, but they speculate that CCDC170 might manage the direction that pigment cells take as they migrate from the future spine line — with those that move sideways producing diamonds, and those that move outward and tailward at the same time creating chevrons.
Ultimately, the anoles use Turing periodicity plus an extra mechanism: the option to spread the pigment cells tailward and create something different. Instead of just Turing patterning, it’s Turing-plus.
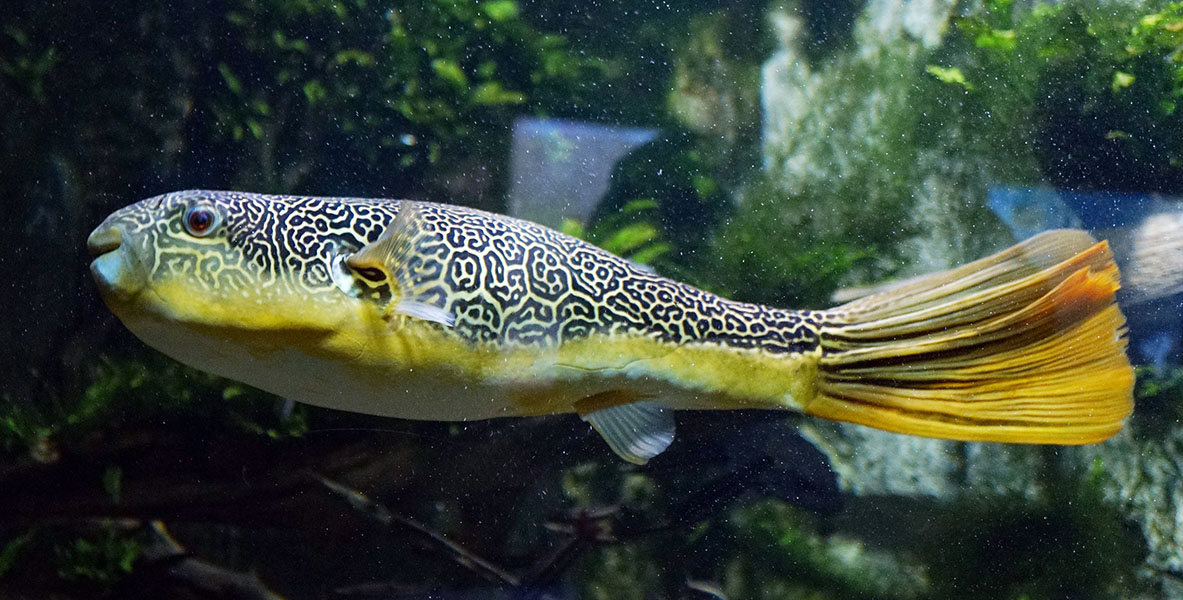
Some fish have complex Turing patterns that look like labyrinths.
CREDIT: TIIA MONTO / WIKIMEDIA COMMONS
Some fish have hit upon another way to complexify Turing spotting, by mixing two versions of the same type of Turing pattern together — call it Turing-squared. Computer models predict that a mashup of black spots on a white background plus white spots on black should create a mazelike distribution of black-and-white lines and curlicues.
In fact, many such labyrinthine fish exist in nature. Miyazawa in Japan analyzed thousands of fish species for spotted and mazelike patterns. In fish families where there are species with both kinds of spotted patterns, there is often a labyrinthine version too. Presumably, these fish wearing maze fashions reflect what the math predicts, a cross between dark spots on light and light spots on dark.
Scientists are also investigating how a creature’s pattern gets laid down early in development. In many cases, developing animals first lay down a colorless prepattern — like the lines in a coloring book. Later on, pigment cells come along to fill in the colors. Cats can serve as prime examples, thanks to the work of Greg Barsh, a developmental geneticist at Stanford University, and colleagues. Cat breeding by people has created an astonishing variety of looks — striped and spotted tabbies, color point Siamese, “ticked” Abyssinians with alternating bands of color on each strand of fur, and so on. In 2012, by examining the skin of developing felines such as tabby housecats and the boldly splotched king cheetah, the researchers began to suss out the elements of the prepattern. They reported that a prepattern is laid down in felines well before pigment cells arrive on the scene.
When those pigment cells finally arrive, there’s only one kind — one “crayon” — that shows up in mammals. It’s called the melanocyte and it deposits pigment in skin or hair cells. Depending on factors such as the signals received by the melanocyte, it can make two kinds of pigments that yield either shades of black/brown or yellow/red. A lack of pigment produces white.
The team recently took their work further, identifying a gene called Dkk4 that seems to produce a Turing Inhibitor; it’s turned on in the skin of fetal cats before any coloring-in takes place.
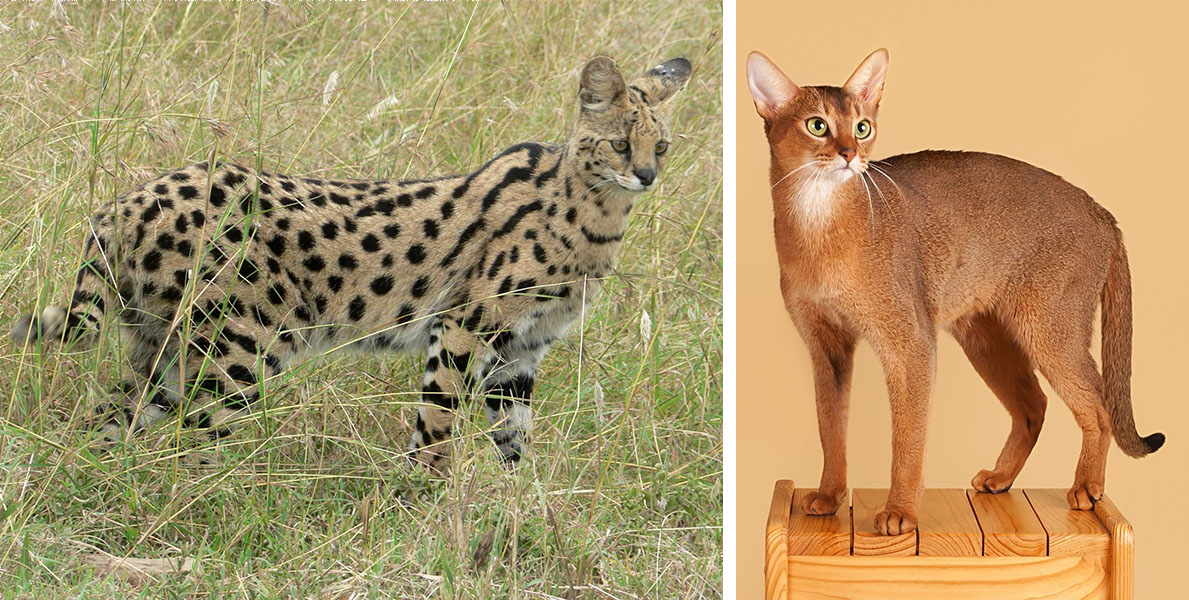
A wild serval cat (left) and an Abyssinian (right).
CREDITS: BUDGIEKILLER (LEFT) OLEG ROYKO (RIGHT) / WIKIMEDIA COMMONS
Genetic studies of adult cats of varied patterns suggest that Dkk4 normally acts to promote wide stripes. Here’s the evidence: Wild servals, found in Africa, have two normal copies of the Dkk4 gene, and they have large, clear stripes and spots, like a tabby. Felines with one normal and one mutant Dkk4 gene — and so a 50 percent dose — have small, numerous spots. And housecats with two broken versions of Dkk4, such as Abyssinians, have ticked fur. Thus, Barsh and colleagues suggest that the Abyssinian’s ticks are really super-thin tabby stripes squished tightly together on each strand of fur.
The protein produced from Dkk4 and related proteins often work in conjunction with another group of proteins, those in the Wnt family. The pair have been linked to Turing patterning in a system not directly related to color: In mice, interactions between Wnts as activators and Dkks as inhibitors lead to evenly spaced hair follicles in the developing skin.
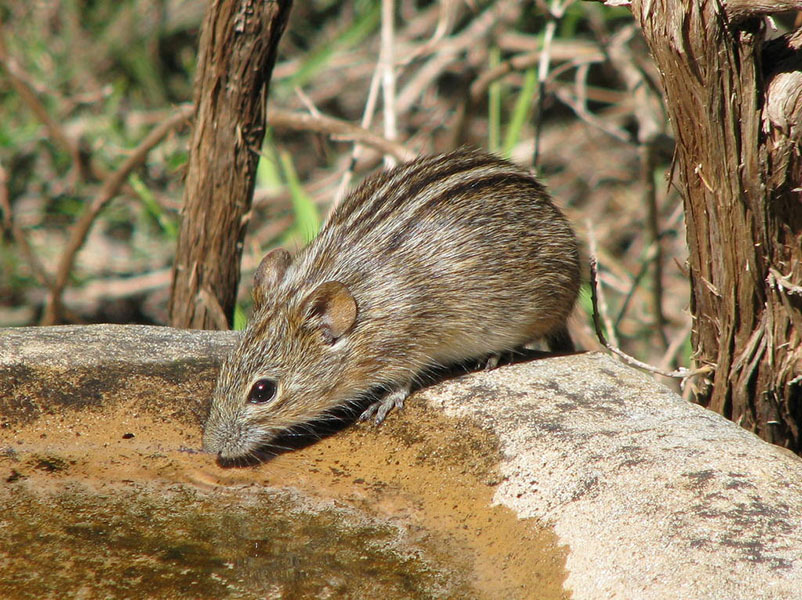
The African striped mouse serves as a convenient system in which to study striping.
CREDIT: MARIAN OLIVER / iNATURALIST
The African striped mouse also seems to rely on Wnt and Dkk proteins, as well as other players, to outline its chipmunk-like racing stripes. Developmental biologist Ricardo Mallarino and colleagues at Princeton University discovered that the striped mouse’s prepatterning — and the chipmunk’s, too — is a result of a Turing system overlaid with something else: in this case, a simple gradient of molecules that are present in high amounts at the spine and lower amounts toward the belly.
Mathematicians had long predicted that the overlay of a simple gradient on top of Turing spotting would generate alternating strips of dense or sparse specks. Imagine a pond with evenly spaced lily pads (the specks), and then drop a rock in the middle. The single wave (the gradient) coming out from the rock would create ripples, with most, but not all, of the lily pads settling in the valleys of the ripples. In biological systems, the math predicts that Turing spotting plus a gradient would, similarly, generate stripes with lots of specks alternating with stripes that have few specks.
In the skin of the developing African striped mice, those lily pads are like the specks where hair follicles will appear — thanks to Wnt proteins. The specks show up first in the areas that will become light stripes, and two days later, in areas destined to be dark. That distribution is created not by a falling rock but by that added gradient, a waning concentration of several Wnt-regulating proteins from spine to belly. The discovery in the striped mice was the first living example of this Turing-plus-gradient pattern that mathematicians had long predicted, says Mallarino.
That’s how the striped mouse makes the prepattern — the coloring-book lines. The colors themselves are the result of another gene that manages how the melanocytes mature: Some get stuck in arrested development and aren’t able to make pigment, so they create light stripes. The ones that do mature fill in the dark stripes.
Turing’s ideas have staying power, even decades after he proposed them. But he didn’t have all the information, and evolution layered complexity over his simple activators and inhibitors.
“The Turing pattern is definitely important,” says Yipeng Liang, a biologist at the University of Virginia in Charlottesville. But, he adds, “nature is more complicated than we thought.”
10.1146/knowable-052324-2
TAKE A DEEPER DIVE | Explore Related Scholarly Articles
