The fluid inside a living cell bustles with activity. Proteins, RNA, lipids and other molecules wiggle, zip, glide and drift through this broth — catalyzing reactions, activating receptors, relaying messages, marking viruses and other foreign molecules for destruction and performing a gazillion other tiny but crucial tasks. It all adds up to keep cells — and the life forms they’re a part of — running smoothly.
Biologists have studied these cellular processes for decades. They know an immense amount about the membrane-enclosed organelles inside cells — mitochondria, endoplasmic reticulum, Golgi bodies and more. And they have dissected, often down to atomic-level detail, some of the molecular machinery that drives biological events.
But at the crucial in-between scale, a big question mark remains: How do the right proteins organize themselves in a sea of fluid swarming with millions of molecules? Do they bump into each other by chance, or does the cell actively organize its fluid space to bring the correct partners together?
The latter appears to be true, according to recent research at the intersection of physics and biology. Over the last decade, cell biologists have come to appreciate what many believe to be a whole new way that cells shape their internal landscape. Like blobs merging, then dispersing, in a lava lamp, or a salad dressing that separates into bubbles of oil and vinegar, groups of proteins can sometimes congeal into distinct droplets. One key way these droplets form is through a process called liquid-liquid phase separation.
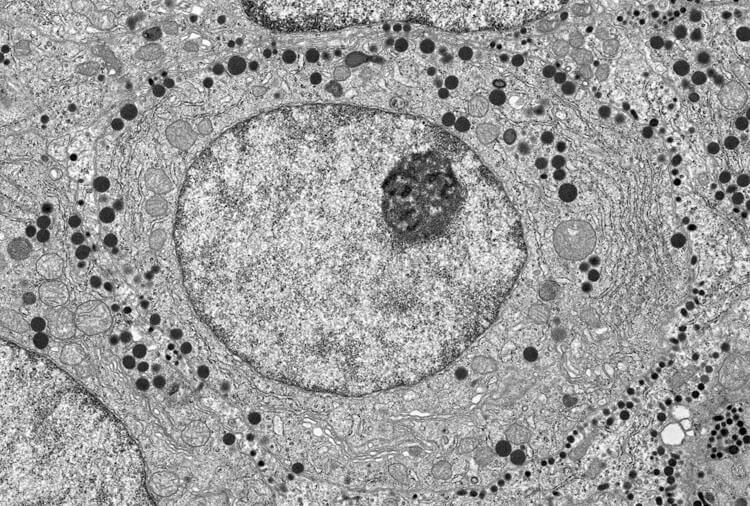
Cells are full of organelles, seen here in a micrograph of a pituitary cell from a rat. Some organelles are enclosed by membranes, such as the nucleus that holds genetic material (large circle filling center of image) and mitochondria that power the cell (pale, circular structures outside nucleus). Other essential work in the cell seems to take place in phase-separated droplets, such as the nucleolus where ribosomes are made (dark circle in upper right of nucleus).
CREDIT: © H. JASTROW
Exactly what happens within these droplets largely remains a mystery. The blobs might act as temporary breakout spaces for specific cellular events, for example. Or their formation might result from a stage in some key cellular process. But whatever their precise functions turn out to be, some biologists think that their formation promises to fundamentally reframe our understanding of how the cell does its essential business.
“This is the revolution that we’ve been waiting for,” says D. Allan Drummond, a biochemist and molecular biologist at the University of Chicago.
From oozy to gooey
Liquid-liquid phase separation is a relatively new concept for cell biologists, notwithstanding a few observations of liquid droplets in cells over the years, including one from more than a century ago. But in the physics world it’s old news — which is handy. “The power of this is that it sits on almost 100 years of condensed matter physics,” says biophysicist Alex Holehouse of Washington University in St. Louis, who coauthored a review in the Annual Review of Biophysics on how phase-separated droplets form.
To understand the concept, it helps to understand the properties of the cell’s interior, or cytoplasm. Researchers call it a fluid, but it’s more oozy than watery, like cornstarch mixed with a bit of water. That’s because it’s chock-full of dissolved molecules, explains Stephanie Weber, a cell biologist at McGill University in Montreal. When clusters of those molecules begin to separate out, they create even gooier, molasses-like pockets of cytoplasm called biomolecular condensates.
Structurally, the proteins within a condensate are a bit like a tangle of cooked spaghetti, if you can imagine spaghetti strands made of weak Velcro. They bind lightly to many parts of the other proteins in the condensate, in no particular orientation. (Contrast that with the key-in-a-lock kind of binding that occurs when an enzyme attaches to a target or a chemical sticks to a receptor.) Many of the proteins or protein regions that make these weak connections are what biochemists call disordered, meaning they don’t take on a firm three-dimensional shape like most proteins do. The sum of all those weak forces holds the droplet together.
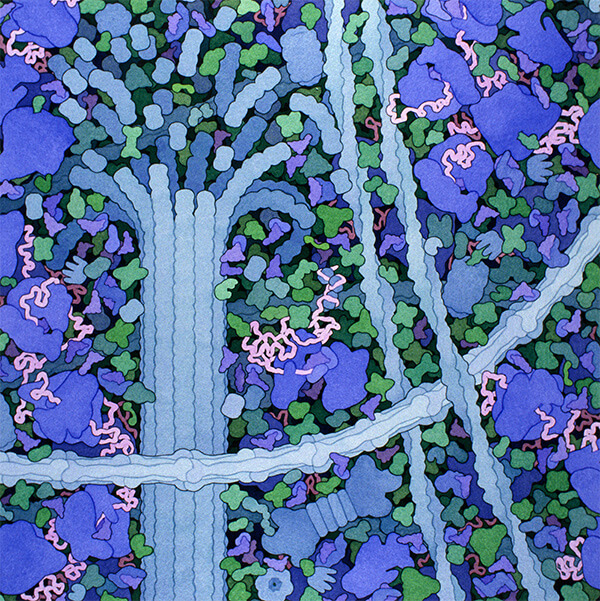
The cytoplasm of a cell is a crowded place. This illustration shows three different components of the cell’s internal skeleton (the blue, rope-like structures), many of the protein-building factories called ribosomes (purple) and numerous other proteins, all crowded together.
CREDIT: ILLUSTRATION BY DAVID S. GOODSELL
Biologists started paying attention to the phenomenon with a study published in 2009 in Science by molecular biologist Anthony Hyman of the Max Planck Institute for Molecular Cell Biology and Genetics in Dresden, Germany, and his colleagues. Hyman and Clifford Brangwynne, then a postdoc in the lab, had attached a green fluorescent dye to individual proteins that form clumps called P granules inside fertilized egg cells of roundworms. The researchers captured on video the green P granule blobs as they transitioned between two different liquid phases — disappearing as they dissolved into the cytoplasm and reemerging as they condensed again. Scientists had known about P granules for decades but had never before described this slithery behavior in terms of dissolving and condensing.
Soon after, a team led by biophysicist Michael Rosen at the University of Texas Southwestern Medical Center reported that proteins in a test tube could undergo phase separation to form droplets. The study showed that this phenomenon, which physicists and chemists have observed in many different molecules, occurs in proteins that can bind many targets.
These studies laid the foundation for a new field. But pinning down what, exactly, such droplets do has been a challenge. “There are a bunch of hypotheses,” Weber says. “But there is relatively little direct evidence for them.”
Goody bags
The biggest, broadest hypothesis for the function of these droplets is that they concentrate specific sets of proteins and other molecules so as to house, kick-start or speed up the reactions the proteins engage in.
For example, concentrating certain proteins in droplets near the cell membrane intensifies signals to assemble the cell’s cytoskeleton, the mesh of filaments that gives a cell its 3-D shape, as Rosen’s team reported in 2019. The phase separation may rev up a molecular process that normally ticks over barely above idle, the researchers proposed.
And work from geneticist Richard Young’s lab at the Massachusetts Institute of Technology suggests that phase separation concentrates droplets of proteins needed to turn on the activity of genes or prod a chromosome to start copying itself at the correct places on the DNA strand. Rather than relying on chance for the right proteins to appear where they are needed, the droplets form what Young calls “a goody bag” of all the components that are necessary for these processes to occur.
Much of the evidence for this and other functions of phase separation is circumstantial so far, because direct proof is tough to get. Researchers generally test how cellular processes work by perturbing them, but it’s hard to disrupt phase separation without also breaking up protein interactions that are closely associated with it, making it challenging to draw conclusions about cause and effect.
Until recently, Drummond worried that the idea of condensates as compartments was distracting researchers from thinking more broadly about what these structureless structures might be doing, though recently their focus has expanded. “There are many alternatives to the simplistic notion that this is all about encapsulating some biochemical reaction,” he says. For example, droplets might keep enzymes or other molecules out of the general cytoplasm so that they don’t undergo certain reactions, releasing them only when the cell needs them or when a process they might interrupt is completed.
Or, as Drummond’s own work suggests, some proteins may phase-separate in response to an environmental cue, such as temperature. In that scenario, a cell’s ability to detect the change might serve as a finely tuned sensor.
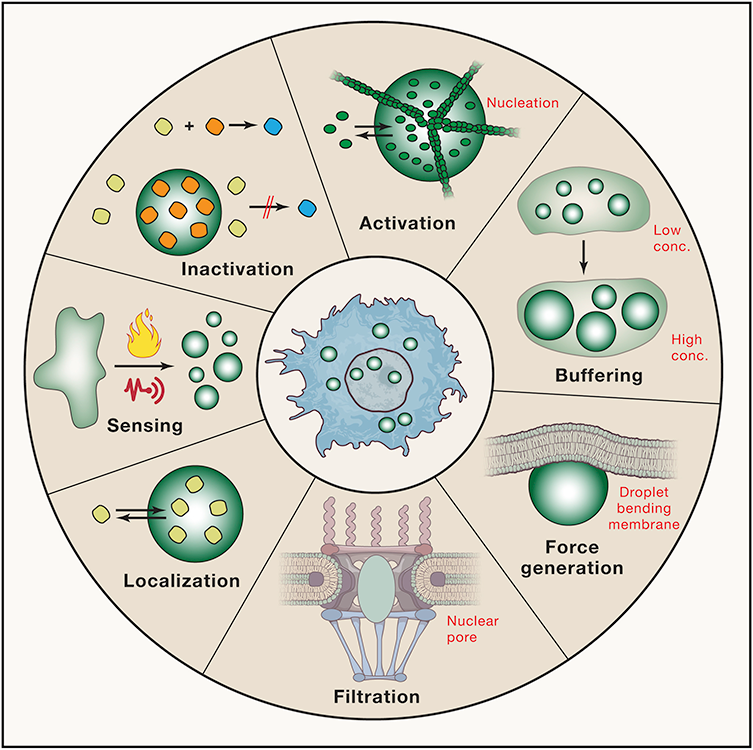
Scientists don’t fully understand what role phase-separated droplets play within cells, but they have some ideas. Clockwise from top, the droplets might activate reactions by concentrating the right molecules in one place; store excess molecules so that the rest of the cytoplasm has the correct concentration; generate mechanical forces that can do work in the cell; act as filters by changing the size of pores, such as in the nuclear membrane; localize particular molecules where they are needed by other organelles; act as sensors by forming under certain physical conditions; or prevent reactions from happening by sequestering molecules that would otherwise react.
CREDIT: S. ALBERTI ET AL / CELL 2019
Whatever functions phase separation might serve, cells seem to suffer when it goes awry. For example, some proteins that aggregate in the brain cells of people with neurodegenerative diseases, such as proteins called FUS in amyotrophic lateral sclerosis, normally exist in phase-separated droplets called stress granules in stressed cells. Introducing mutations that have been linked to ALS into FUS proteins causes the granules to solidify more readily, which can be harmful, says Hyun Kate Lee, a biochemist at the University of Toronto. Aggregates in other brain diseases, such as tau in Alzheimer’s disease or alpha-synuclein in Parkinson’s disease, also appear to be caused by phase separation run amok, Lee says. She and others are exploring ways to reduce aggregation of these abnormal droplets.
Other diseases also appear to be linked to phase separation — most notably cancer, perhaps because of the process’s role in turning genes on and off. Structural biologist Tanja Mittag’s lab at St. Jude Children’s Research Hospital in Memphis found that mutations in a cancer-linked gene called SPOP interfere with phase separation. That may, in turn, drive the cancer, perhaps by leaving molecules that are usually safely sequestered in condensates to roam free.
Here, too, it may be possible to target condensates to fight the disease, Young says. He and his colleagues recently reported that in breast cancer, certain condensates take up concentrated amounts of chemotherapy drugs that target tumors. If many or most drugs concentrate in particular droplets, this could dramatically affect the way new drugs are developed and tested, he says.
Mittag and others agree that they have barely scratched the surface of how this new field might transform cell biology. “I think there’s no doubt that phase separation plays a very important role for cells,” she says. Researchers so far have identified at least 20 different types of phase-separated droplets, each consisting of different proteins and other molecules and emerging under different circumstances.
Some condensates, like P granules, are long-standing characters in the cell, newly identified as products of phase separation. Others are just emerging. The diversity is not surprising, says Lee: Just like cell organelles that are bounded by membranes all have different functions, membraneless ones probably do too.
“There’s no question that these things are exciting and there’s tons to figure out,” Drummond says. “It is new biology, and that doesn’t come about very often.”