Where the heck did all those structures inside complex cells come from?
Scientists agree that eons ago, a bacterium took up residence inside another cell and became its powerhouse, the mitochondrion. But there are competing theories about the birth of other organelles such as the nucleus and endoplasmic reticulum.
Support sound science and smart stories
Help us make scientific knowledge accessible to all
Donate today
More than 1.5 billion years ago, a momentous thing happened: Two small, primitive cells became one. Perhaps more than any event — barring the origin of life itself — this merger radically changed the course of evolution on our planet.
One cell ended up inside the other and evolved into a structure that schoolkids learn to refer to as the “powerhouse of the cell”: the mitochondrion. This new structure provided a tremendous energetic advantage to its host — a precondition for the later evolution of complex, multicellular life.
But that’s only part of the story. The mitochondrion is not the only important structure within complex, eukaryotic cells. There’s the membrane-bound nucleus, safekeeper of the genome. There’s a whole system of internal membranes: the endoplasmic reticulum, the Golgi apparatus, lysosomes, peroxisomes and vacuoles — essential for making, transporting and recycling proteins and other cargo in and around the cell.
Where did all these structures come from? With events lost in the deep past and few traces to serve as evolutionary clues, it’s a very tough question to tackle. Researchers have proposed various hypotheses, but it is only recently, with some new tools and techniques, that cell biologists have been able to investigate the beginnings of this intricate architecture and shed some light on its possible origins.
A microbial merger
The idea that eukaryotes originated from two cells merging dates back more than 100 years but did not become accepted or well known until the 1960s, when the late evolutionary biologist Lynn Margulis articulated her theory of endosymbiosis. The mitochondrion, Margulis said, likely originated from a class of microbes known as alphaproteobacteria, a diverse group that today includes the bacterium responsible for typhus and another one important for the genetic engineering of plants, among many others.
Nothing was known about the nature of the original host cell. Scientists proposed that it already was fairly complicated, with a variety of membrane structures inside it. Such a cell would have been capable of engulfing and ingesting things — a complicated and energetically expensive eukaryotic feature called phagocytosis. That might be how the mitochondrion first got into the host.
But this idea, called the “mitochondria late” hypothesis, doesn’t explain how or why the host cell had become complex to begin with.
In 2016, evolutionary biologist Bill Martin, cell biologist Sven Gould and bioinformatician Sriram Garg, at the University of Dusseldorf in Germany, proposed a very different model known as the “mitochondria early” hypothesis. They argued that since no primitive cells today have any internal membrane structures, it seems very unlikely that a cell would have had these over 1.5 billion years ago.
Instead, the scientists reasoned, the endomembrane system — the whole hodgepodge of parts found inside complex cells today — could have evolved soon after the alphaproteobacterium took up residence inside a relatively simple host cell, of a kind from a class called archaea. The membrane structures would have arisen from bubbles, or vesicles, released by the mitochondrial ancestor.
Free-living bacteria shed vesicles all the time, for all sorts of reasons, Gould, Garg and Martin note, so it seems reasonable to think they’d continue to do that when enclosed inside a host.
Eventually, these vesicles would have become specialized for the functions that membrane structures perform today inside eukaryotic cells. They would even fuse with the host cell’s membrane, helping to explain why the eukaryote plasma membrane contains lipids with bacterial features.
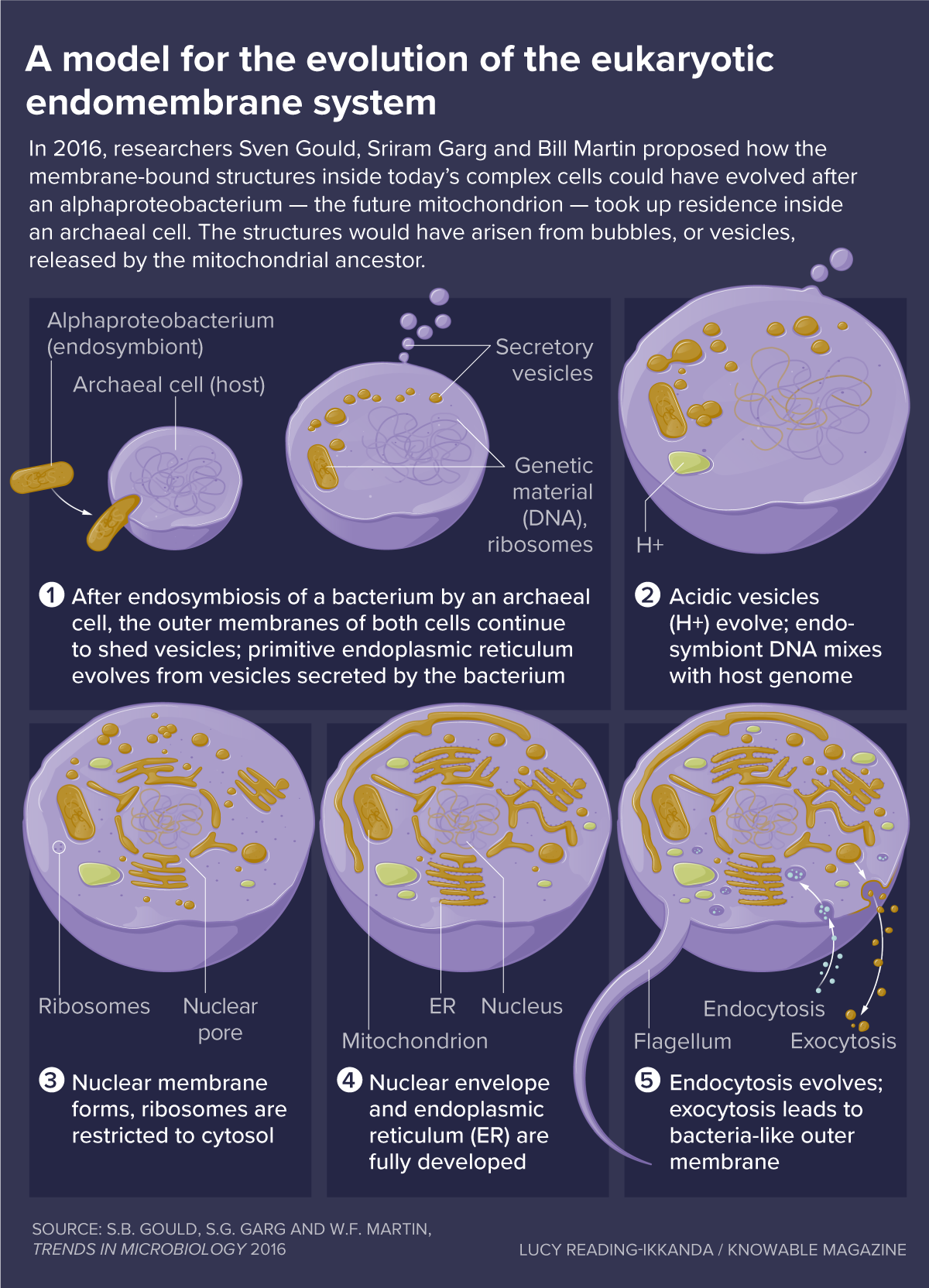
Vesicles could have served an important initial function, says biochemist Dave Speijer of the University of Amsterdam. The new endosymbiont would have generated plenty of poisonous chemicals called reactive oxygen species, by oxidizing fatty acids and burning them for energy. “These destroy everything, they are toxic, especially on the inside of a cell,” Speijer says. Sequestering them inside vesicles would have helped keep the cell safe from harm, he says.
Another problem created by the new guest could also have been helped by making membranes barriers, Gould, Garg and Martin add. After the alphaproteobacterium arrived, bits of its DNA would have mixed with the genome of the archaeal host, interrupting important genes. Fixing this would mean evolving machinery to splice out these foreign pieces — today they’re known as introns — from the messenger RNA copies of genes, so those protein-making instructions wouldn’t be garbled.
But that created yet another problem. The protein-making machinery — the ribosome — works extremely fast, joining several amino acids together per second. In contrast, the intron-removing system of the cell is slow, snipping out about one intron per minute. So unless the cell could keep the mRNA away from ribosomes until the mRNA was properly processed, the cell would produce many nonsensical, useless proteins.
The membrane surrounding the nucleus provided an answer. Serving as a spatial barrier, it allows mRNA splicing to finish up in the nucleus before the intron-free mRNA is translated in the cell’s internal fluid, the cytosol. “This is the selective pressure behind the origin of the nucleus,” Martin says. To form it, vesicles secreted by the endosymbiont would have flattened and wrapped around the genome, creating a barrier to keep ribosomes out but still allowing small molecules to pass freely.
An inside-out explanation
In short, Gould, Garg and Martin’s hypothesis explains why endomembrane compartments evolved: to solve problems created by the new guest. But it doesn’t fully explain how the alphaproteobacterium got inside the host to begin with, says cell biologist Gautam Dey at EMBL in Heidelberg, Germany; it assumes the endosymbiont is already inside. “This is a massive problem,” Dey says.
An alternative idea, proposed in 2014 by cell biologist Buzz Baum of University College London (with whom Dey once worked) and his cousin, University of Wisconsin evolutionary biologist David Baum, is the “inside-out” model. In this scenario, the alphaproteobacterium and the archaeal cell destined to be its eventual host would have lived side by side for millions of years in an intimate symbiosis, each depending on the other’s metabolic products.
The archaeal cell would have had long protrusions, as seen on some modern-day archaea that live in close association with other microbes. The alphaproteobacterium would have nestled up against these slender extensions.
Eventually, the protrusions would have wrapped around the alphaproteobacterium and enclosed it completely. But during the long stretch of time before that happened, the archaeal cell would have begun some spatial division of labor: It would keep information-processing jobs in its center, where the genome was, while functions like protein building would take place in the cytosol within the protrusions.
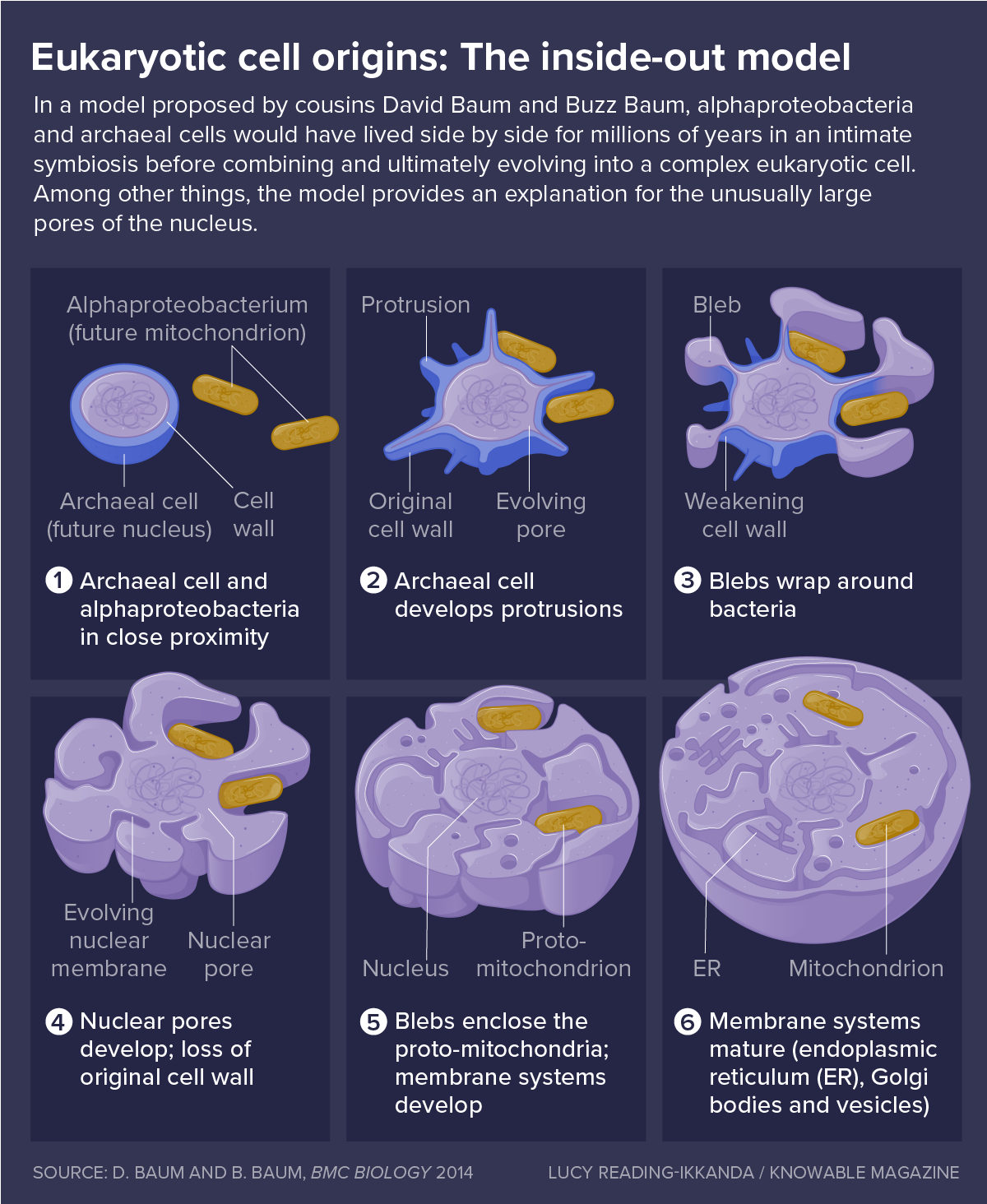
The power of the inside-out model, Buzz Baum says, is that it gives the cell eons of time, before the alphaproteobacterium becomes fully enclosed, to evolve ways to regulate the number and size of the mitochondrion and other membrane compartments that would eventually become fully internal. “Until you can regulate them, you’re dead,” Buzz Baum says.
The model also explains why the nucleus has the shape that it does; in particular, it provides an explanation for its unusually large pores. Viewed from inside the center of an archaeal cell, the long protrusions would be openings that could naturally become big pores like those, Baum says.
Most important, the inside-out model explains how the alphaproteobacterium would have gotten inside the archaeal host in the first place.
Still, the inside-out model has features it needs to explain. For example, the mitochondrion would end up in the wrong place — inside the endoplasmic reticulum, the network of tubes on which sit the cell’s protein-making ribosomes, as the archaeal protrusions wrapped around it. And so an additional step would be required to get the alphaproteobacterium into the cytoplasm.
But Martin’s main objection is that the inside-out model does not provide an evolutionary pressure that would have caused the nucleus or other membrane-bound compartments to arise in the first place. The inside-out model “is upside-down and backwards,” Martin says.
The nucleus: A riddle in the middle
Though the models agree that the mitochondrion evolved from an alphaproteobacterium, they have very different ideas about the origin of the nucleus and other organelles.
In the Gould, Garg and Martin model, the source for all of the structures would have been vesicles released by the evolving mitochondrion. Vesicles to contain reactive chemicals or cellular cargo, and the ability to move this cargo around, would have evolved very early. The nucleus would have come later.
In the inside-out model, the nucleus was, essentially, the remains of the archaeal cell after it wrapped its membranes around the alphaproteobacterium. So it would have appeared immediately. The endoplasmic reticulum also would have formed early, created from those squished-together protrusions. Other organelles would have come later — arising, Buzz Baum says, from buds of archaeal membrane.
Thus the models also make different predictions about the chemical nature of the membranes of cell organelles — at least originally — and how today’s complex cells came to have membrane lipids that are all chemically like the ones in bacteria, not archaea.
In the Gould, Garg and Martin model, in the beginning all the membranes except for the host cell’s outermost one would have been bacterial, like the membranes of the new resident. Then, as bacterial vesicles fused with this archaeal outer membrane, the bacterial lipids would slowly replace the archaeal ones.
In the inside-out model, the membranes of the nucleus and endoplasmic reticulum — and probably others — would have been archaeal, like the host, to start. Only later on, after genes from the bacterial genome moved over to the archaeal genome, would the lipids become bacterial in nature, Baum suggests.
How to test these ideas? Through experiments, cell biologists are starting to glimpse ways in which simple vesicles could have diversified into different organelles with distinct jobs — by taking on different shapes, like the layered membrane stacks of the modern endoplasmic reticulum or the Golgi body, or by ending up with different proteins inside them or on their membranes.
They are also highlighting the dynamism of the modern-day mitochondrion — and its potential to spawn new membrane structures.
Take, for example, the compartment that Speijer thinks evolved early in order to deal with reactive oxygen species: the peroxisome.
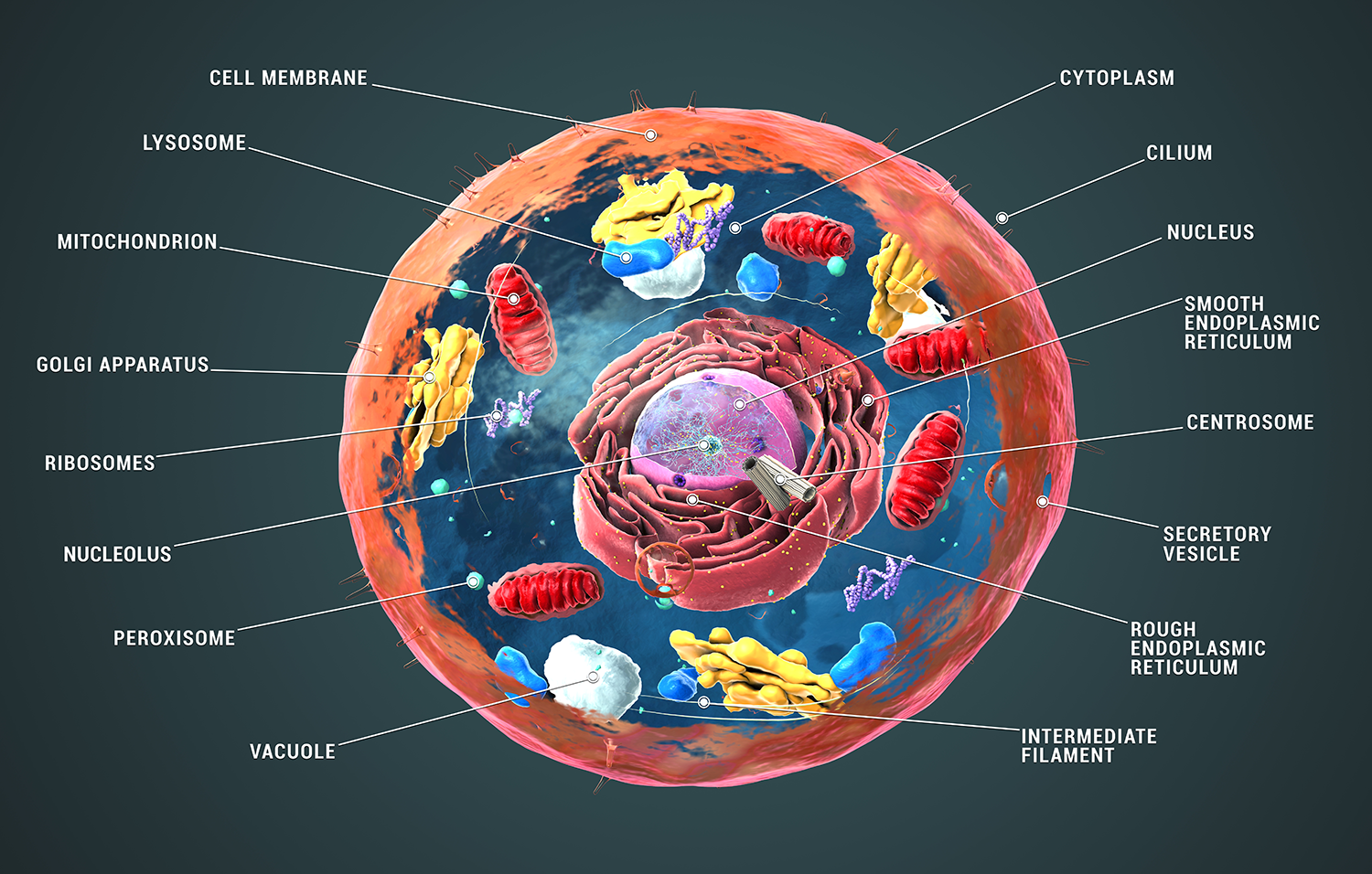
Modern eukaryotes have many specialized cell compartments that carry out different jobs. This spatial and functional specialization distinguishes them from their prokaryotic forebears.
CREDIT: ISTOCK.COM / CHRISTOPH BURGSTEDT
In 2017, cell biologist Heidi McBride of McGill University in Montreal reported that cells lacking peroxisomes could generate them from scratch. Working with mutant human fibroblast cells without peroxisomes, her team found that these cells put proteins that are essential for peroxisome function into mitochondria instead. Then the mitochondrial membrane released them as little bubbles, or vesicles.
These vesicles, or proto-peroxisomes, matured into true peroxisomes when they fused with another type of vesicle derived from endoplasmic reticulum, which carry a third necessary peroxisome protein. “It’s a hybrid organelle,” McBride says.
For McBride, this is an indication that peroxisomes — and probably other organelles — originally came from mitochondria (not exclusively from the endoplasmic reticulum, as previously believed). “The presence of mitochondria launched the biogenesis of new organelles,” she says. “In the case of peroxisomes, it’s quite direct.”
Other mitochondrion antics have also been noted.
First, a 2021 report from the lab of biochemist Adam Hughes at the University of Utah found that when yeast cells are fed toxic amounts of amino acids, their mitochondria will shed vesicles that are loaded with transporter molecules. The transporters move amino acids into the vesicles, where they won’t poison the mitochondria.
Hughes also discovered that the vesicles shed by the mitochondria can form long, tubule-like extensions with multiple layers, reminiscent of the layered stacks of the endoplasmic reticulum and the Golgi body. The structures persist in the cell for a long time. “They’re definitely their own unique structure,” Hughes says.
And in 2022, immunologist Lena Pernas, now at UCLA, showed that multilayered, mitochondria-derived structures can form in other contexts, too. When a cell is infected by the parasite Toxoplasma, her team found, the mitochondria surround the parasite and change shape. The parasite responds, and the upshot is that the mitochondrion ends up shedding large bits of outer membrane.
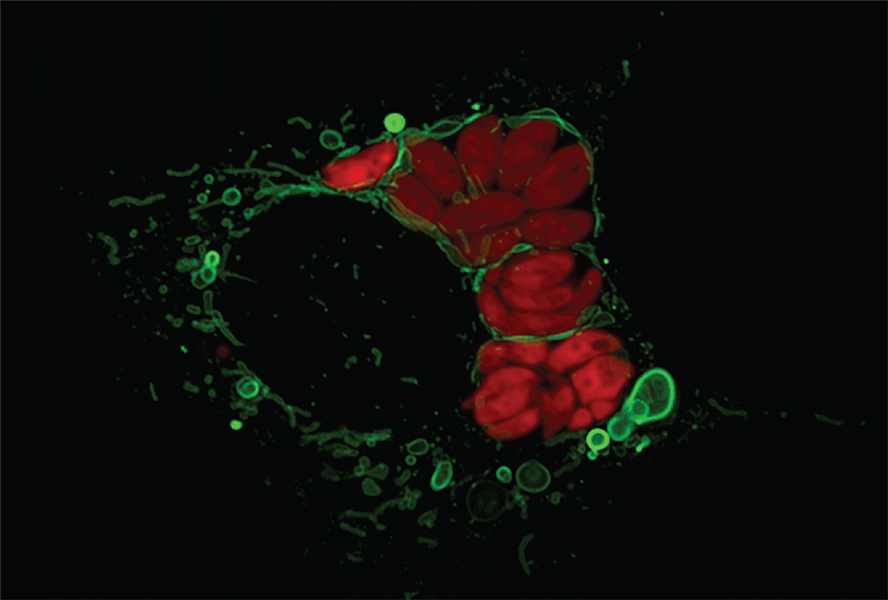
This microscopic image shows what happens when the parasite Toxoplasma gondii (in red) infects a mouse cell. The cell’s mitochondria (in green) gather around the invader and begin to shed vesicles (green bubbles) made of mitochondrial outer membrane. Sometimes, the vesicles can form elaborate, multilayered structures. Observations like this suggest a way in which the various endomembrane structures could have evolved early on during the evolution of eukaryotes.
CREDIT: LENA PERNAS
Pernas, who wrote about mitochondrial remodeling in the Annual Review of Physiology in 2016, recently discovered that these structures, which initially look like simple vesicles, also can grow and take on more complex shapes, such as stacks of sheet-like layers. What’s more, the stress of infection changes what sorts of proteins are placed on these shed bits of mitochondrial membrane. Such changes open the door for the stacked sheets to behave in different ways than they normally would, presenting the opportunity to take on new jobs, Pernas says.
The more Pernas and Hughes study these structures — found in quite different cells and conditions — the more similar they look. It’s tantalizing, says Hughes, to imagine how a structure like this, forming in the early days of eukaryote evolution, could have evolved over eons of natural selection into some of the endomembrane compartments existing in cells today.
It may never be possible to know for sure what happened such a very long time ago. But by exploring what can happen in today’s living bacterial, archaeal and eukaryotic cells, scientists can get more clarity on what was possible — and even probable. A cell moves into another cell, bringing benefits but also problems, setting off a complex cascade. And then, McBride says, “all this stuff blooms and blossoms.”
10.1146/knowable-102323-2
TAKE A DEEPER DIVE | Explore Related Scholarly Articles
