These proteins have been secretly managing your cells
Scientists have long known that histones spool DNA and help regulate genes. They may be doing a lot more.
Support sound science and smart stories
Help us make scientific knowledge accessible to all
Donate today
Every second, as we breathe, sleep, eat and go about our lives, millions of biochemical reactions are happening in our cells. Among the hurly burly of chemical exchanges are ones that attach small carbon molecules onto (or remove them from) proteins, fats, DNA and more. Adding or taking away these small molecules is essential for many reactions that enable cells to survive, grow and divide.
Perhaps the most interesting and well-studied target of these additions and subtractions lies within the bustling nucleus, where various enzymes attach or remove two small molecules — methyl groups and acetyl groups — onto histones, the protein spools around which our DNA is wrapped.
For decades, adding or removing methyl or acetyl groups to histones was thought to be key to when and where genes are turned on.
But accumulating evidence shows that this is only part of the story. Although putting methyl and acetyl groups on histones is closely linked with activity of nearby genes in some places in the genome, in many other regions it has no impact at all. This suggests that regulating gene activity is not the only function of these histone decorations — perhaps not even the main one.
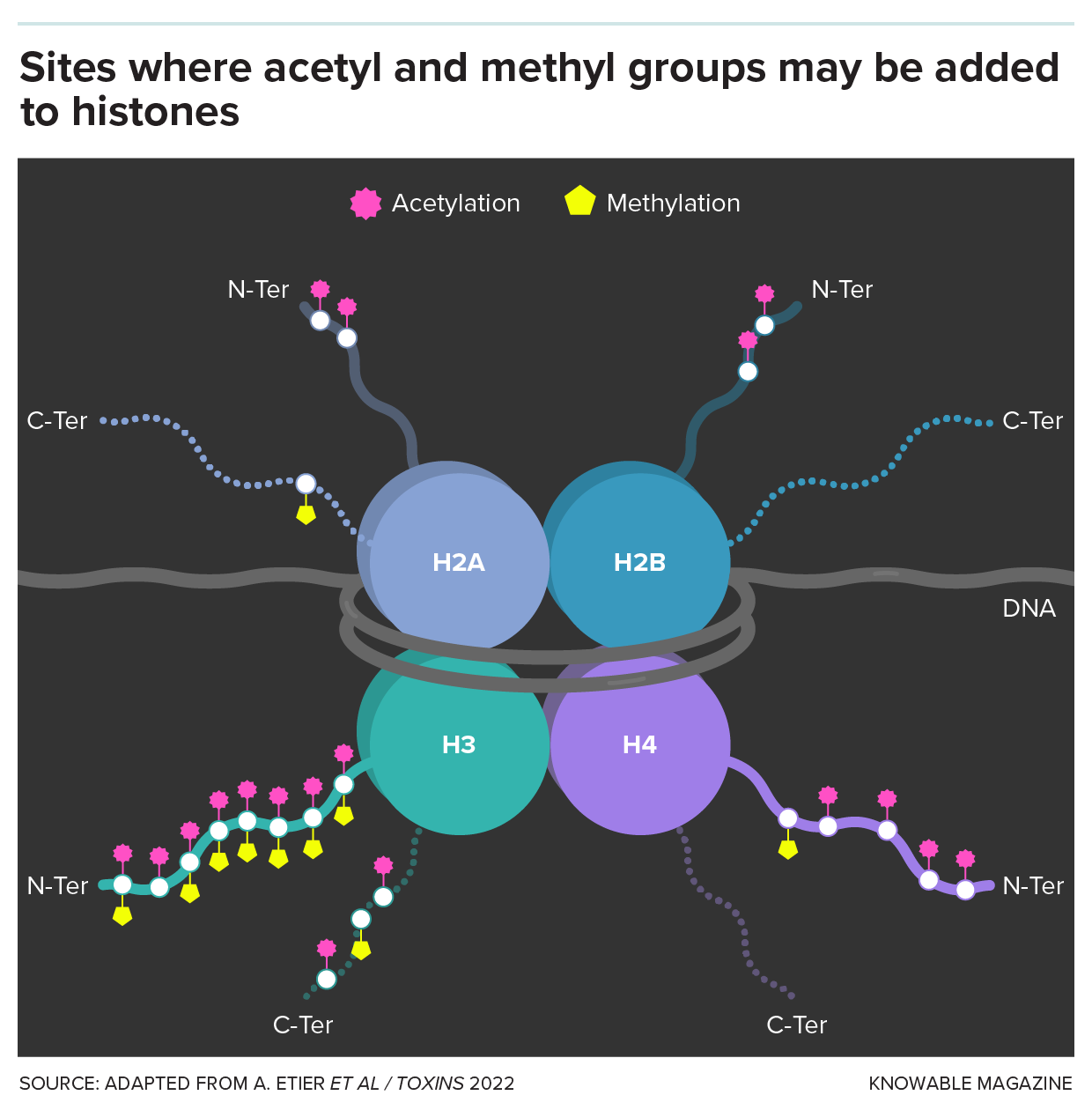
The histone proteins of complex cells are named H2A, H2B, H3 and H4. They are assembled into groups of eight, around which a length of DNA is wrapped. This structure, called a nucleosome, repeats along the length of a chromosome. Histones have long tails onto which metabolites like acetyl and methyl groups can be added by enzymes.
In fact, emerging research suggests that these modifications to histones have key roles in the cell’s biochemical processes — its metabolism — functioning as a way for the cell to deal with small carbon molecules that are produced during biochemical reactions.
Researchers propose that for acetyl groups (made of two carbons, three hydrogens and one oxygen), the histones serve as a kind of bank or repository that the cell can draw on when it needs more acetyls for chemical reactions.
And for methyl groups (one carbon atom and three hydrogens), they suggest that the histones serve as sinks, where methyls can be put so they don’t gum up chemical reactions. Without this sink, many molecules that need to lose a methyl group to proceed to the next step in a biochemical pathway stall out, causing problems for the cell.
Histones were once viewed as mere structural scaffolding for genes: something that could keep dense folds of DNA strands in order. Then they were seen as involved in gene control — either facilitating or blocking the unfolding of DNA that enables it to be copied. Now, if the new research pans out, they will also prove to be deeply intertwined with the metabolic workings of the cell.
And this, scientists say, may help to reveal how and why histones evolved in the first place.
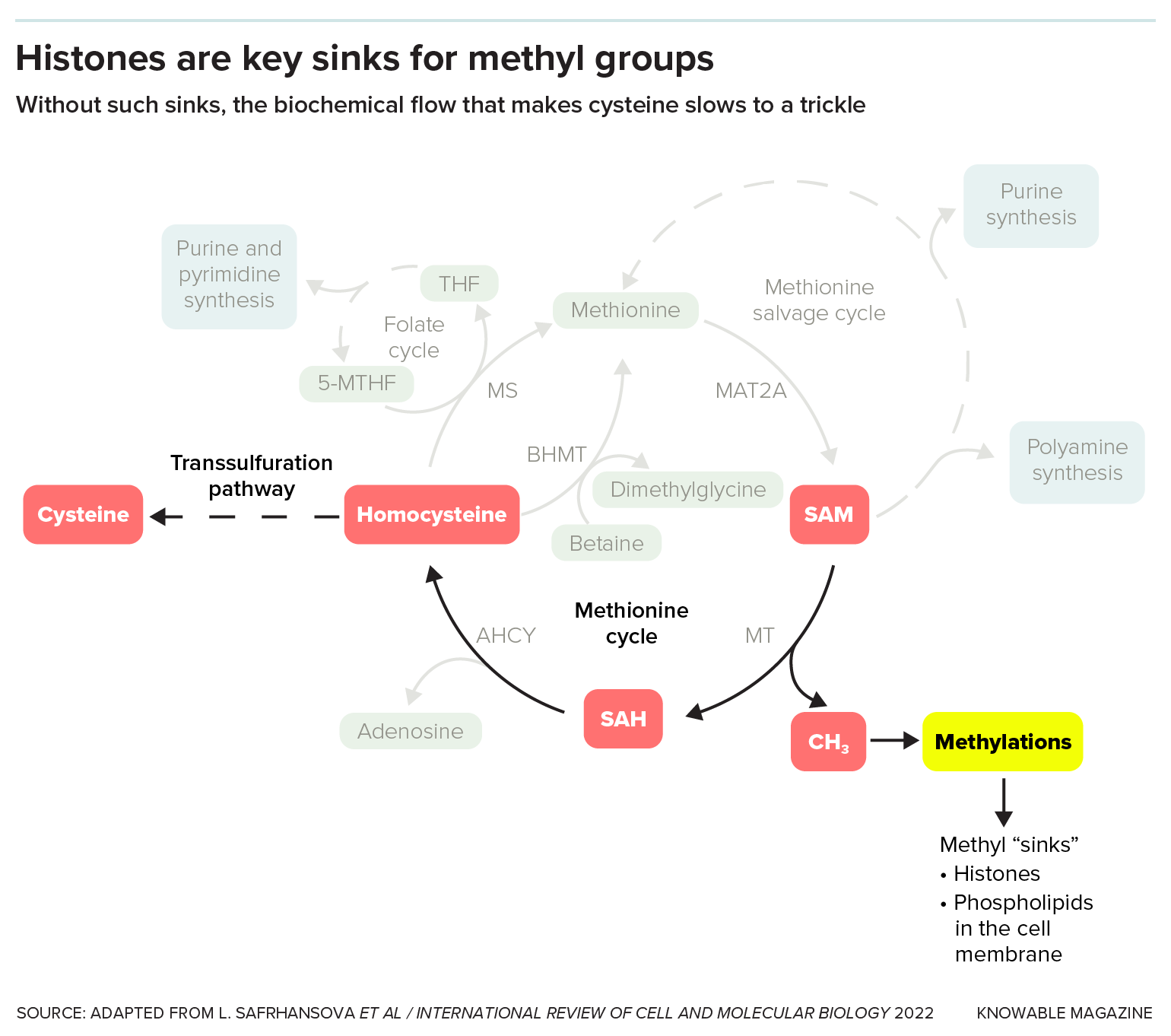
Cells need places to deposit methyl groups — a methyl sink — so that metabolism does not gum up. In this diagram, a chemical called SAM donates a methyl group to a methyl sink, allowing the biochemical pathway for making the amino acid cysteine to proceed. If SAM is prevented from donating its methyl group, the pathway slows to a trickle and the result is a cysteine deficiency in the cell. Researchers propose that histones act as these important sinks.
Signaling times of plenty
More than a decade ago, UT Southwestern biochemist Benjamin Tu was growing yeast cells in his lab when he saw something interesting: The activity of more than a thousand genes was oscillating in step with how much oxygen the cells were consuming. Gene activity and metabolic activity were changing in a coordinated way.
Tu also saw that when genes involved in cell growth were at their peak activity, this coincided with high numbers of acetyl groups stuck on their histones. And when the genes went silent in the next phase of the cell cycle, the acetyl groups went away. “That was very exciting,” Tu says.
It was exciting because acetyl groups are produced by the mitochondrion — the cell’s power-generating organelle. Acetyl groups are used by the cell to make molecules like fatty acids that are used for energy or to build cell membranes. What seemed to be happening was that acetyls were serving as a signal from the mitochondrion to the cell nucleus that these were times of abundance, with lots of available energy and chemical building blocks. By sticking onto the histones, they were ramping up activity of genes involved in cell growth. It makes sense, after all, to grow and divide during times of plenty.
Tu also saw signs that the acetyls on histones could also act as a bank — a source of energy for the cell to draw on when times became leaner. When cells were starved, he observed, the amount of an important chemical called acetyl-CoA — which is central in energy generation — decreased in the cell. To make energy, the cells consumed acetyl groups that had detached from the histones. The acetyl groups that remained were rearranged so that they would activate genes to produce more acetyl-CoA.
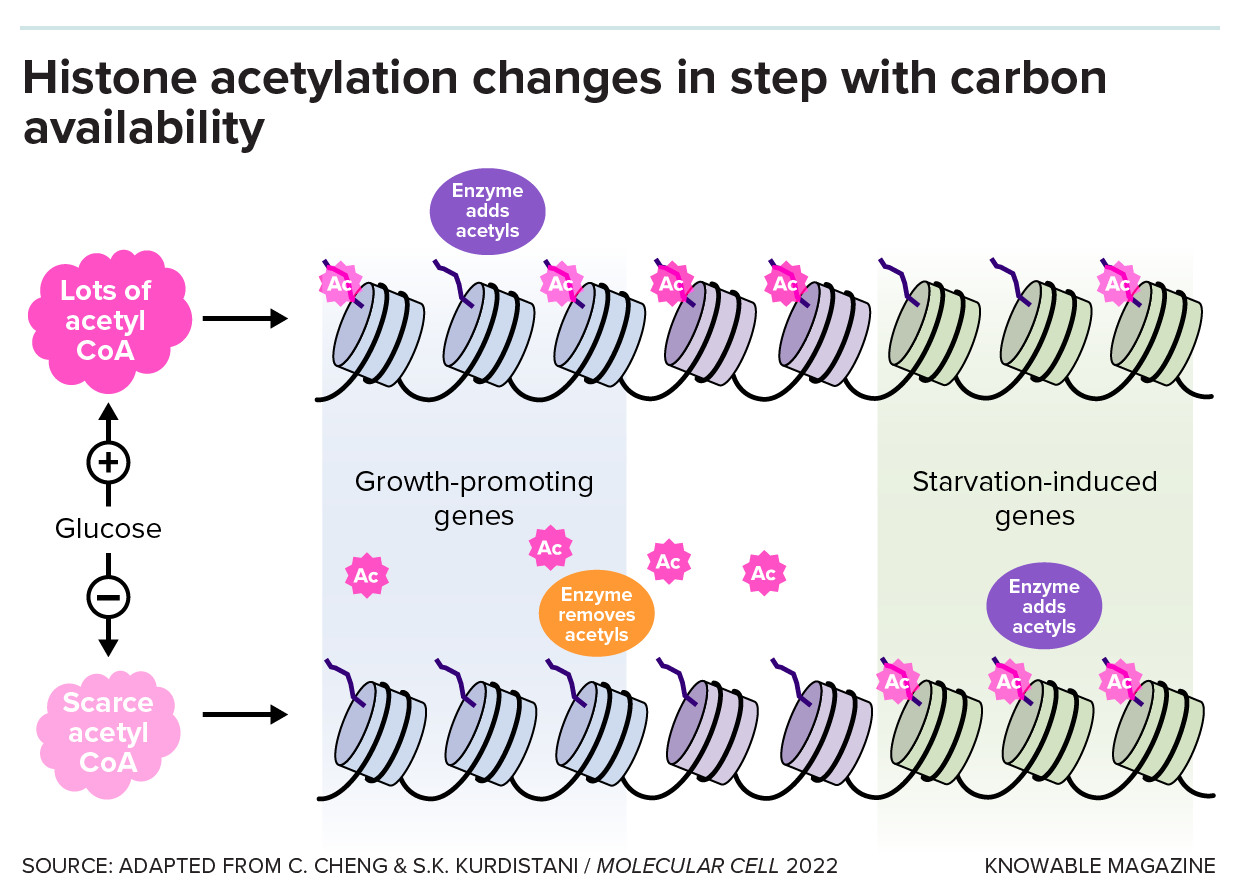
This illustration depicts a model for the movement of acetyl groups during different cell conditions. When energy is abundant and mitochondria are running at full speed, acetyl groups are plentiful and become attached to histone tails on genes that promote growth. When food becomes scarce, the cell consumes these acetyl groups for energy. The remaining acetyl groups are redistributed to activate genes involved in increasing amounts of acetyl CoA in the cell.
Other work by Tu’s group suggests that histones may play an even more central role in metabolic pathways, this time involving methyl groups. Again in yeast, the scientists studied a chemical that carries methyl groups around — its name is SAM for short. When SAM gives up a methyl group, it turns into a chemical that is needed to make the amino acid cysteine. But when the cell doesn’t have a place to give up its methyl groups to, too little cysteine gets made, affecting the cell’s ability to grow. Histones serve as these methyl group receivers.
Keeping metabolism humming
More evidence for a metabolic role of histones comes from a 2023 study in which University of Oxford biochemist Peter Sarkies and his colleague Marcos Francisco Pérez examined a whole host of different enzymes that all add methyl groups to histones.
Each enzyme puts methyl groups on at a unique place on the histone — a floppy part called the histone tail. Depending on where the methyls are added, the effect can be associated with activated gene activity, suppressed gene activity or no change at all. Sarkies reasoned that, if one is simply trying to get methyl groups out of the way so that metabolism can proceed, what matters is the sum activity of all of these enzymes –– not any individual enzyme or a particular effect on a nearby gene.
This is exactly what his team saw when they examined a number of cancer cell lines. Each cell line had raised or lowered the activity of different combinations of those methylating enzymes, so they could deposit methyl groups on histones to tuck them out of the way and keep metabolism proceeding apace.
The scientists also found that many of the methylating enzymes were under the influence of a gene called Rb known for its role in suppressing cancer (it is often mutated in cancer cells). This suggested to Sarkies that Rb plays a central role in increasing or decreasing the rate at which methyl groups are deposited on histones and thus regulating biochemical pathways and growth.
“What we discovered is that the cell uses histone methylation not just to regulate genes, but to regulate metabolism,” Sarkies says.
Yet more possibilities
Researchers have also recently learned that histones can sometimes involve themselves in other aspects of cell biochemistry. In a study published in 2017, chromatin biologist Marcus Buschbeck’s team at the Josep Carreras Leukaemia Research Institute in Barcelona showed that a type of histone called macroH2A1.1 can help to preserve a chemical called NAD+, which is essential in many biochemical reactions. This leaves more NAD+ around for the power-generating mitochondria to use.
And University of California, Los Angeles, biochemist Siavash Kurdistani’s team showed in 2020 that histones function as enzymes that convert oxidized copper ions (Cu2+) to reduced copper ions (Cu1+). Reduced copper ions are the form required by the mitochondria to produce energy. These ions would have been hard to come by at the dawn of the evolution of complex, eukaryotic cells, like our own, because copper was oxidized as levels of oxygen rose in the atmosphere.
As they uncover this histone-metabolism link, researchers are also speculating on how the relationship emerged to begin with.
They note that in microbes called archaea — from which eukaryotic cells are thought to have evolved — there is a great variety of histones. But very few of these have the floppy tails that our own histones have, onto which the methyl and acetyl marks are placed. So scientists are interested in understanding how histones may have functioned in our archaeal ancestors.
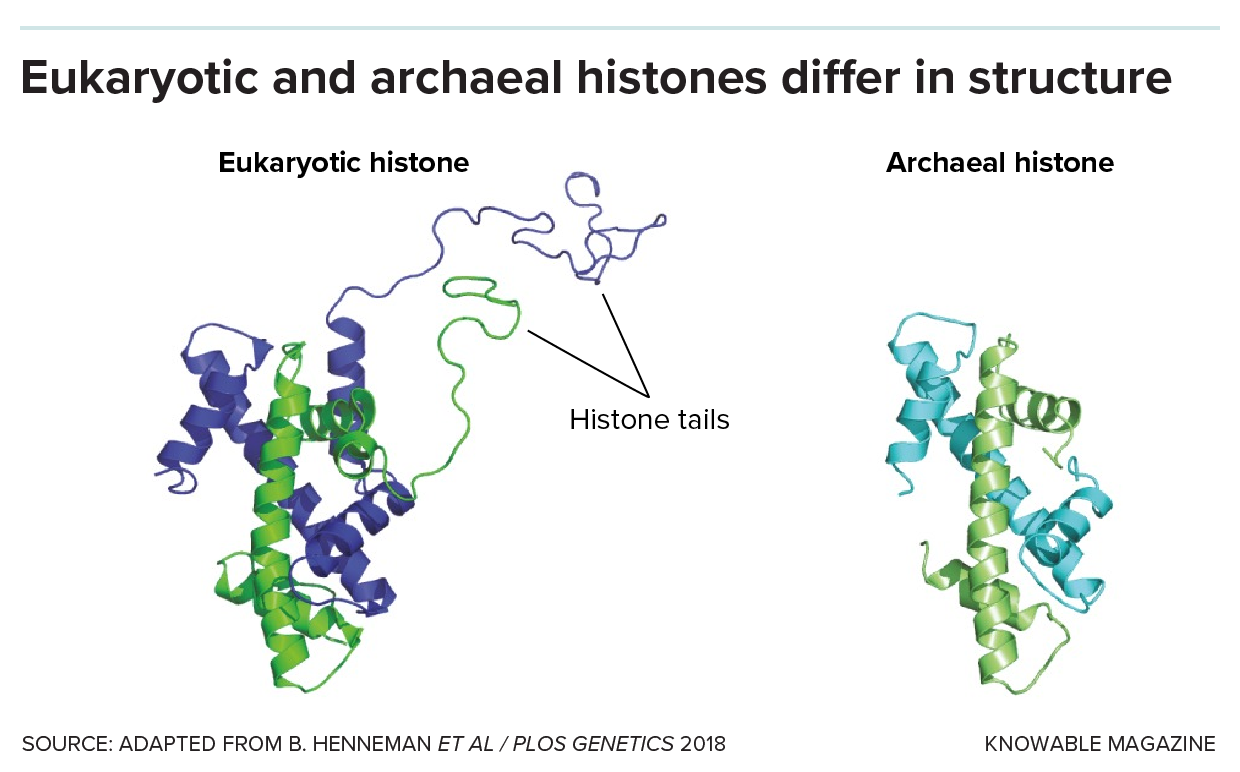
Most histones of single-celled life forms called archaea have no tails or short tails. But the histones of eukaryotic cells like our own have long, floppy tails on which the cell attaches various metabolites.
They float a variety of possibilities. Kurdistani suggests that the early role of archaeal histones could have been to produce those precious reduced copper ions. Chromatin biologist Tobias Warnecke of Imperial College in London, who studies the evolution of histones in archaea, suggests that archaeal histones could help to keep DNA from breaking apart in the extreme environments such as high heat that archaea live in. Histones might also have protected the archaeal DNA from viruses seeking to insert themselves into it, Warnecke adds.
Later on, after the ancestor of today’s eukaryotes appeared some 1.5 billion years ago, the histones evolved longer tails that were chemically modified in a variety of ways, including by acetyl and methyl groups. It’s possible, Tu says, that such modifications arose to manage the metabolites produced by the mitochondria in those early eukaryotes. Some chemicals produced in the mitochondria are highly reactive and could spontaneously stick to — and damage — important molecules like DNA. Maybe the cell evolved enzymes to remove these small carbon molecules from places where they would be harmful and stick them instead in places like histone tails where they would cause no harm.
Later on, the cell could have become dependent on these modifications of histones for their metabolic regulation.
And later still? The story of the evolution of histones appears to be one of repurposing. If cells first stumbled into a way to regulate their metabolism with histones, Sarkies says, a similar process could have led to using them to control genes. For histones, he suggests, “metabolic regulation is more fundamental than gene regulation.”
10.1146/knowable-082824-1
TAKE A DEEPER DIVE | Explore Related Scholarly Articles